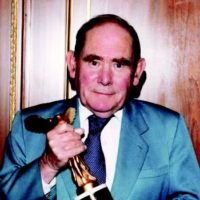
Sydney Brenner
Molecular Sciences Institute
For 50 years of brilliant creativity in biomedical science — exemplified by his legendary work on the genetic code; his daring introduction of the roundworm Caenorhabditis elegans as a system for tracing the birth and death of every cell in a living animal; his rational voice in the debate on recombinant DNA; and his trenchant wit.
Sydney Brenner likes beginnings. In the course of his career, he has opened up several major fields. While many scientists are gearing up to explore the new frontiers he has pioneered, Brenner's brain is already fidgeting and scouting around for a fresh path.
In the early 1960s, Brenner emerged as one of the influential leaders of the classical period of molecular biology, the era from 1953 (discovery of the double helix) to 1966 (elucidation of the genetic code). His discoveries are legendary and appear in every biology textbook. He established the existence of messenger RNA (mRNA) — the 'working tape' copy of genetic material from which cells make proteins — and found that each amino acid in a protein is specified by a set of three building blocks, or nucleotides, in the RNA chain, which he termed "codon." He showed that the nucleotide sequence in mRNA dictates the order of amino acids, and that each mRNA chain contains the instructions for a particular protein. He also deciphered the stop codons, which terminate protein synthesis.
Having contributed significantly to the budding field of molecular genetics, he abandoned it in favor of a new adventure in the middle 1960s. “Once we understood about DNA, the code, and making proteins, a lot of detail had to be filled in, but we thought that most of the important problems had been solved,” he says. “We could leave it to the hordes of other people to continue. We wanted to do something more interesting.”
He turned to embryonic development and the nervous system. In seeking an organism to study, he wanted an animal in which he could map the complete wiring of the nervous system and make mutations to study the function of individual genes. He chose the roundworm Caenorhabditis elegans. This organism is composed of a fixed number of cells, each of which performs the same task from individual to individual. Furthermore, each cell in the developing organism gives rise to a predictable set of cells in the adult, offering the possibility of building a flow chart of each cell’s fate.
Brenner figured out how to work with C. elegans in the lab. Because the animal is a hermaphrodite, making mutants requires procedures that differ from those employed with, for example, fruit flies. Using these techniques, Brenner and postdoctoral fellow John Sulston (now at the Sanger Centre in England) set to work unraveling how C. elegans grows and specializes on its trajectory from a single cell to a full-grown worm.
The researchers found that some cells kill themselves during embryonic development. By exploiting the relative ease of probing the roles of single genes in C. elegans, postdoctoral fellows such as Robert Horvitz (now at MIT) began untangling the molecular mechanism that underlies this controlled cell suicide, also called apoptosis. That work opened the door to understanding this phenomenon, which plays a critical role in many organisms’ embryonic development and also guards against cancer. Brenner and postdoctoral fellows in his lab have exploited C. elegans to launch the study of many additional complex physiological phenomena, including aging, nerve cell function, and transmission of chemical signals from the outside of a cell to its inside.
The group also elucidated the detailed wiring of the nervous system, the first and thus far only such diagram for any animal. The researchers cut the worms into literally thousands of thin slices and viewed them with an electron microscope. By stacking up the resulting cross-sectional pictures, they could trace each neural connection. With this method, they uncovered exactly how nerve cells link to each other in the entire animal.
Brenner’s team also started to pinpoint where particular genes lay on the worm’s six chromosomes. This work gave rise to the C. elegans genome project, spearheaded by former Brenner postdoctoral fellows Sulston and Robert Waterston (now at Washington University School of Medicine), in which scientists spelled out the complete sequence of the organism’s DNA. This endeavor, completed in 1998, produced the first whole genome sequence of an animal.
In these ways, Brenner elevated this obscure worm from the pages of zoology books to a central place in experimental biology. “There are maybe one thousand people working in C. elegans now,” he says. “Things really took off.”
Brenner studied C. elegans until the mid-1980s. By that time, “There were lots of talented young people who could do things better than I could,” he recalls. Furthermore, he’s most interested in new fields, he says, making a chess metaphor: “The exciting thing about science is the opening game. C. elegans is in the middle game. I thought I would like to do something different.”
So he switched to study genomes of other organisms, and started working on the human genome. In 1986, he thought up the then-novel approach of focusing on DNA sequences that encode proteins (as opposed to the vast stretches that have no known function). This approach funnels sequencing efforts toward the parts of the genome that represent the working components of the cell. He then spurred the UK to employ this method.
Having made his mark on the human genome project, Brenner turned to the pufferfish, an organism with its genes organized compactly. “It has no junk DNA,” he says. “In one-eighth the size of the human genome, it’s got all of the genes.” Because many pufferfish genes substitute effectively for mouse genes, comparing the regions shared by pufferfish and mouse genes can reveal which parts are most important: those sequences that evolution has left unchanged. “What appeals to me is that, in a sense, the DNA contains a historical record of the organism,” he says. “You can reconstruct the past with contemporary sequences.” The goal is to untangle how organisms evolved from one another, and he is currently tackling this task.
In addition to ferreting out interesting scientific challenges, Brenner has made significant contributions to keeping the gears of inquiry running smoothly. During the early days of recombinant DNA research, as investigators were developing the ability to cut and paste together different pieces of DNA and transfer genes from one organism to another, some people feared the creation of dangerous new breeds of microbes. As a result, several governments declared a moratorium on these types of experiments.
Eventually, the UK decided that the work could proceed, but the scientists had to take steps to prevent the microbes from escaping from the labs into the outside environment. Brenner devised a completely different solution to the potential problem. “They were thinking about physical containment,” he says, “But you could have biological containment: you could work with disabled strains.” Such a version of the microbes wouldn’t survive well outside the lab, he reasoned.
To prove that this approach would work, Brenner took an unconventional tack and tested some genetically weakened bacteria on himself. “I drank them,” he says. Always a rigorous experimentalist, “I measured how much of the disabled strain came through my intestines.” It survived very poorly compared to the bacteria that normally inhabited his body.
He points out that this technique of transferring genes from one organism to another allowed people to work safely with dangerous germs such as HIV. A viral genome is much safer in a bacterium such as Escherichia coli than in its normal viral packaging. “It took people a long time to realize that cloning it in E. coli makes it safer rather than more dangerous,” says Brenner.
Underlying Brenner’s interest in molecular genetics, embryonic development, the nervous system, genomes, and evolution is his curiosity about how the internal description of an organism — its genes — generates a fully functioning living thing. This topic — at the heart of biology — differs from all other sciences, he says. “It’s a very particular subject because the changes are made in the gene script, but they’re meaningless at that level. They have to be run in the organism and tested in the world.” He founded a multidisciplinary research organization, The Molecular Sciences Institute, in 1996 to probe these issues through a combination of genomic biology research and computation and simulation.
Brenner presents his views about biology with humorous analogies, some of which he has detailed in a series of columns that appear as a regular feature in Current Biology. In one of these, the co-discoverer of the DNA double helix, Francis Crick, throws up his hands in frustration at failing to understand how the fruit fly’s wings develop from an embryonic organ. “God knows how these imaginal discs work,” cries Crick, according to Brenner. Because only God knows, Crick ends up visiting him and asking for an explanation. Brenner envisions God’s response: “Well,” comes the reply, “We took a little bit of this stuff and we added some things to it and… actually, we don’t know, but I can tell you that we’ve been building flies up here for 200 million years and we have had no complaints.”* Unlike other complex engineering feats, building a living organism does not follow a strict recipe. Flexibility, in fact, plays a central role in organisms’ evolution.
Brenner comments on scientific culture as well as concepts, conjuring up fanciful scenes to illustrate his points. One column leads into a discussion about how science differs from country to country, and ends with a whimsical illustration of how audiences in different places respond to a seminar speaker who takes off his pants mid-speech. In England, the audience sits impassively; in Germany, everyone rises and removes their trousers; in France, a uniformed guard asks the speaker to leave; in Italy, the pants get stolen.
Brenner has woven a colorful and richly textured scientific tapestry from threads of intense curiosity, imagination, intellect and determination. His legacy reaches into many areas of inquiry, and promises to stretch well into the future.
by Evelyn Strauss
*S. Brenner. (1996). “Francisco Crick in Paradiso.” Curr. Biol. 6, 1202.
Key publications of Sydney Brenner
Brenner, S., Jacob, F., and Meselson, M. (1961). An unstable intermediate carrying information from genes to ribosomes for protein synthesis. Nature. 190, 576–581.
Crick, F.H.C., Barnett, L., Brenner, S., and Watts-Tobin, R.J. (1961). General nature of the genetic code for proteins. Nature. 192, 1227–1232.
Jacob, F. and Brenner, S. (1963.) Genetique Physiologique—sur la regulation de la synthese du DNA chez les bacteries: l’hypothese du replicon. C.R. Acad. Sci. 256, 298–300.
Brenner, S., Stretton, A.O.W., and Kaplan, S. (1965). Genetic code: the nonsense triplets for chain termination and their suppression. Nature. 206, 994–998.
Brenner, S. (1974). The genetics of Caenorhabditis elegans. Genetics. 77, 71–94.
Brenner, S., Elgar, G., Sandford, R. Macrae, A., Venkatesh, B., and Aparicio, S. (1993). Characterization of the pufferfish (Fugu) genome as a compact model vertebrate genome. Nature. 366, 265–267.
Award presentation by Joseph Goldstein
Groucho Marx may hold the distinction of being the funniest comedian who ever lived, but Sydney Brenner, this year’s recipient of the Lasker Special Achievement Award, holds a loftier title — the funniest scientist who ever lived. Like Groucho, Sydney is famous for his flamboyant energy, his creative one-liners, and his insightful monologues on every subject imaginable — not to mention his bushy eyebrows. Fifty years ago, Groucho Marx taught Americans the meaning of the Yiddish word chutzpah when he refused membership in a Beverly Hills country club because he didn’t care to join any club that would elect him as a member. Like Groucho Marx, Sydney Brenner is the personification of chutzpah.
What other scientist would have the chutzpah to present a lecture at Harvard wearing a splashy Hawaiian shirt?
What scientist would have the chutzpah to submit a manuscript to the Royal Society with a fake reference tucked away in the middle of the text, “Leonardo da Vinci (personal communication)”? And when called on the carpet by the editor, fired back that there’s a new Italian postdoctoral fellow working in the lab.
What scientist would have the chutzpah to refuse a knighthood from the Queen of England? I doubt that even Groucho Marx would have the chutzpah to refuse the club at Buckingham Palace.
Francis Crick describes Sydney Brenner in much the same way: “Sydney is unique, both in his characteristic sense of humor that colors his many unusual insights and in the breadth and importance of his biological discoveries. There is simply nobody else quite like him.” This is high praise from the High Priest of Biology.
So who is Sydney Brenner and what are his scientific achievements that place him in the company of Picasso, Stravinsky, and Groucho Marx? It is intimidating to attempt to summarize in several minutes the 50-year career of a scientific giant like Sydney Brenner. It may not be possible, but let me try. This will be a real act of chutzpah on my part!
Like everything else about Sydney, his early life was unusual. He was born in 1927 in a small town outside of Johannesburg, South Africa — far removed from the academic centers of the world. His parents had emigrated to South Africa in 1910 from Latvia and Lithuania. His father was a shoemaker who worked every day until he was over 80. His father could neither read nor write, but he could speak multiple languages. Despite these humble beginnings, Sydney taught himself to read the newspaper by age three. By age four, he had become a voracious reader. By age six, he had discovered the adult section of the public library, where he read his first chemistry books. By age 10, he was doing chemistry experiments at home, extracting pigments from leaves and petals. His brilliance was obvious to everyone, and he soon won a scholarship to medical school at the University of Witwatersrand in Johannesburg, entering at the tender age of 14.
Histology was his favorite course, and he became fascinated with cells and chromosomes. While a medical student, he read every book he could find on cytogenetics and even built himself a centrifuge so that he could determine the chromosome number of Elephantulus — a tiny mouse-like shrew. Scientists typically learn science by working in the laboratories of scientists. But this was not Sydney’s style: he taught himself science. Who else could possibly teach Sydney? Teaching himself science is a recurrent theme in Sydney’s career, as you’ll hear more about in a moment.
In order to graduate from medical school, students in Johannesburg had to pass an exam in which they had to correctly diagnose an unknown sick patient. In the last year of his six-year curriculum, Sydney was sent off to the bedside of a man with diabetic ketoacidosis and told to smell the patient’s breath. When asked what he smelled, he replied “McClain’s toothpaste!” He failed the exam and had to wait another year to graduate.
This delay worked to Sydney’s advantage in several ways: first, he was only 20 years old, which was below the legal age to practice medicine in South Africa; and second, the extra year gave him the opportunity to continue his cytogenetic experiments and the leisure to read every biology book and journal that he could get his eyes on. He became wildly excited by papers from the United States and Europe describing how bacteriophages could be used to study genes. Sydney sensed the emergence of a new field — soon to be known as molecular biology, which was much more challenging to him than practicing medicine. But there was one major problem. Science in South Africa in the early 1950s was scientifically primitive: there were no PhD programs for Sydney to enter. So in 1952, he left Johannesburg to take a graduate degree in biochemistry at Oxford.
Sydney’s timing could not have been better. He arrived in Oxford a few months after Jim Watson had arrived in Cambridge. According to Watson, he and Sydney “added badly needed doses of genetic fresh air to the British biological world.” When Sydney heard about the double helix, he rushed to Cambridge to see the newly constructed DNA model and to meet the pair of young geniuses who conceived it. At their first meeting, Francis Crick was greatly impressed with Sydney’s quick analytic mind, and he set about to recruit him to the MRC Laboratory at Cambridge. When Sydney joined the MRC staff several years later, there was no space for his office so he moved in with Crick, a temporary arrangement that continued for the next 20 years, much to the benefit of the scientific world.
Between 1954 and 1966, Sydney emerged as one of the handful of heroes who presided over the golden age of molecular biology. With Crick, Sydney deduced the triplet nature of the genetic code and coined the term codon. On his own, he worked out the fundamental nature of mutations, showing that single base changes in DNA lead either to altered proteins or to the absence of proteins — the so-called frameshift, suppressor, and nonsense mutations. With François Jacob, he conceived the idea of messenger RNA, the transcript that conveys the genetic information in DNA to the protein synthesizing machinery of the cell, and he went on to perform ingenious experiments to prove its existence. The discovery of messenger RNA is one of the most thrilling pieces of detective work in the history of biology, involving a dramatic series of twists and turns. The action began at Oak Ridge, Tennessee; moved to the Pasteur Institute in Paris; then to Sydney Brenner’s rooms at King’s College; then to a party at the Golden Helix, Francis Crick’s home in Cambridge; then to Matthew Meselson’s laboratory at Caltech; and culminated in a ‘eureka moment’ when Sydney and François Jacob were relaxing on the beach near Los Angeles. Out of the blue, Sydney sprang up from the sand and screamed out to Jacob: “It’s the magnesium! It’s the magnesium.” For those interested in the unabridged version of this historical event, I refer you to Horace Freeland Judson’s The Eighth Day of Creation and François Jacob’s The Stature Within. For his pioneering work on the genetic code and the discovery of mRNA, Sydney received the Lasker Award for Basic Research in 1971.
In the mid-1960s, Sydney made the daring decision to launch a new program in developmental biology designed to learn how a multicellular animal develops from a single fertilized egg. His Grand Scheme was to find an animal in which he could trace the birth and death of every one of its cells. In typical Brennerian fashion, Sydney read extensively, taught himself zoology, tried many pilot experiments involving a multitude of different organisms, and finally settled on a tiny roundworm that lives in the soil called Caenorhabditis elegans, or C. elegans for short. He chose this particular worm because it was small, translucent, anatomically simple, and suitable for electron microscopy. It was also easy to cultivate in the laboratory and to manipulate genetically. Up to 100,000 worms can be grown in a single Petri dish the size of a silver dollar. Each parent worm gives rise to more than 250 progeny with a generation time of only 3.5 days, much shorter than that of the fruit fly. The appeal of C. elegans lies in its simplicity. Compared to a typical human who has more than a trillion cells, C. elegans has only 959 cells in its entire body. Yet, C. elegans has a range of differentiated cell types and does all the basic things that animals do — it moves, it eats, it defecates, and it has sex.
Once Sydney settled on C. elegans, it took about 10 years to prove to other scientists that useful biology would emerge. By 1974, Sydney had succeeded in isolating and mapping the chromosomal location of 300 mutations that altered the movement or the morphology of the worm. All of this initial work was done by Sydney himself, but he soon began to attract top-flight postdoctoral fellows from around the world. The field grew rapidly, from one scientist 30 years ago to over 2000 today, all of whom are descendants of Sydney — his students, his student’s students, and even great grandstudents.
The development of C. elegans has been scrutinized to a degree unmatched by any other multicellular organism. Since 1974, more than 10,000 genetic variants of C. elegans have been produced in over 100 different laboratories throughout the world. It is the only animal in which the complete lineage and fate of every cell is known. C. elegans is the only animal in which the three-dimensional wiring diagram of the nervous system has been mapped at the electron microscopic level. In 1998, it became the first animal whose genome was sequenced. C. elegans has also proved to be a medical Rosetta Stone, providing clues to cancer, Alzheimer’s disease, and diabetes.
Sydney’s dream for the Grand Scheme has come true. The worm has achieved its place in history, along with the fruit fly and the mouse, as one of the three animal models that are revealing the basic principles of multicellular life.
Sydney’s skills have not been limited to the laboratory. He is as shrewd a politician as he is a scientist. During the early hysteria of the recombinant DNA controversy, Sydney’s was the sane voice that soothed the politicians and scientists who worried that the gene-transformed bacteria would escape from the labs and find their way into public places like Central Park. Sydney made the crucial suggestion to use enfeebled strains of bacteria that could not survive outside the lab. He became convinced that this approach would work after testing genetically crippled bacteria on himself. He drank a cocktail full of disabled bacteria and then measured how much of it came through his intestines. None survived. Now recall that in 1975 human experimentation with recombinant DNA was outlawed. Sydney obtained permission for his experiment by telling the British authorities that the enfeebled bacteria would be tested on “an upper primate.” Now that’s chutzpah!
Sydney Brenner is an indefatigable raconteur who is legendary for his trenchant wit and his marvelous impiety. When asked by the Educations Committee of the British Parliament how much mathematics do students need to learn to do biology, Sydney replied: “The ability to count to 20 — that’s all — there are 4 bases and 20 amino acids. You might have to go to 64 at some stage. There are 64 codons in the genetic code.” Sydney has recently published a collection of his humorous views on biology in a book entitled “Loose Ends,” which I recommend to everyone. It’s loaded with delicious anecdotes.
After 50 years in science, Sydney remains as dynamic as ever. He recently invented an ingenious technique to clone DNA on the surface of microbeads sitting in a test tube. This is a far cry from the traditional method of cloning that involves growing bacteria in an incubator. The basic idea is take a complex mixture of DNA, such as all the genes in the brain, and cut it into one million different fragments. Each fragment is then copied 100,000 times and attached to a single microbead. The result is a library of 1 million microbeads, each one of which contains 100,000 copies of a unique piece of DNA. Since the entire library of 1 million microbeads is contained in one test tube, it can be rapidly probed and sequenced. And in true 21st century fashion, Sydney has started a biotech company (Lynx Therapeutics, Inc.) to bring his invention to the masses.
In the 20 years that Sydney shared an office with Francis Crick, they concocted ingenious schemes to explain the workings of nature. Some of their schemes were more rational than the actual scheme that God chose. The take-home lesson is clear: If Sydney had shared an office with God during the Creation, the Universe would be a much more rational place.
Interview with Sydney Brenner and Errol Friedberg
Errol Friedberg, professor and chair, Department of Pathology, University of Texas Southwestern Medical Center in Dallas, interviews Sydney Brenner, winner of the 2000 Albert Lasker Special Achievement Award. Dr. Brenner is President and Director of Science at The Molecular Sciences Institute in Berkeley, California.
Part 1: Early Education
Growing up in the “relative isolation” of South Africa, Dr. Brenner says he became a self-taught chemist until going on to university there and later to Oxford.
Friedberg: To begin this formally, on behalf of the entire community of past and present molecular biologists, let me extend my congratulations to you on receiving the Special Achievement Award.
This award recognizes your lifetime of contributions to science. Let me start by asking you this: In retrospect, was growing up in the relative isolation of South Africa a good or bad thing for you? And if you were to have your scientific life again, would you do it the same way, or differently?
Brenner: It’s hard to say if it’s good or bad because I can’t do the control experiment. That’s where I grew up, and I think the upshot was that it was good for me. And indeed, if I’d grown up somewhere else, I would have been somebody else. So I think, what it let me do was, of course, to become essentially self-taught. I think lots of people who grew up in South Africa had to exploit their own initiatives in learning about things. So it had that benefit. It inculcated at least one good habit.
Friedberg: How did your interest in science in that part of the world, so early on, evolve to chromosomes and genes?
Brenner: I think like most children, I got interested in nature very early. And then by reading a little book called The Young Chemist by Sherwood Taylor, I got interested in actually doing chemical experiments. So I started my career as a garage chemist, buying chemicals from the local pharmacist and making things according to the prescriptions then, and going forward to more experiments, just on that basis. I then started — still while I was at school — extracting pigments from petals and just doing really cookbook chemistry, on that basis. And of course when I came to university, a whole new realm of biology was opened up to me because in doing first-year medicine studies, I did both zoology and botany. And while I was doing botany, I was able to work for someone (on the faculty). I saw chromatography being practiced, which just taught me how naive I was with my own pigment extractions and partitions. Then I got interested in biochemistry. And I got interested in how are things are determined, of course, through just studying anatomy, physiology, botany and zoology — through biological things. So at that time, I was interested in (what we call) cell physiology. When I started moving towards trying to define something to do research on, I decided that I would work on chromosomes. So that’s how I got into this. And the most formative book at that time was reading Wilson’s The Cell in Development and Heredity.
Friedberg: Then you went to Oxford, and as I recall working with (Cyril) Hinshelwood was not an especially inspiring experience for you.
Brenner: Well, it was. I went to Oxford because I wanted to do something that I thought might be the closest thing to my interest, which I had formulated then as cell physiology. Of course, molecular biology hadn’t been invented yet. And I had been given the chance to work on bacteriophage because he (Hinshelwood) was interested in bacteriophage resistance. And so before I came to Oxford, while I was still in Johannesburg, I read this little book, Viruses 1950, which was my first contact with phage. And that was my first conversion to the whole of that stream, which finally ended up in creating molecular biology. So when I came to Oxford, I had already started to work on phage and bacteria.
And of course, as everybody knows, Hinshelwood was not a great believer in mutation. He thought everything was done (in bacterial cells) by simply (shifting) the balance of the kinetics of reactions amongst the different molecules. And he wrote a book called The Physical Chemistry of The Bacterial Cell, in which he said that one could shift balances (in cells) by intervention of the environment, and then the whole state could become inherited. Now, of course, he was right (to a certain extent. There are states in organisms which are like that, except he chose the wrong subject, the wrong kind of bacteriophage (phenotypes) to investigate. And I think that if you look at how he would have interpreted resistance or virulence (in bacteria), or in fact any of the switches we now know about, like the switch from lysogeny to lysis in a lysogenic bacterium, he was absolutely correct there. But, indeed, you know what I was working on was not in question: These were true phage-resistant mutants. Actually, I think I was able to convince him of that at the end by pointing out to him a phrase I have often liked to use over and over—that there are essential differences between impotence and chastity, although the external behavior could be quite the same.
Part 2: Encounter with Francis Crick
When Brenner met Jim Watson and Crick at Cambridge, he says he realized what his life’s work would be. Brenner and Crick shared an office for some 20-odd years.
Brenner: Well, I think when I came to Oxford in the first term, that is in October of 1952, I met Jack Dunitz and Leslie Orgel. And we started to have discussions about DNA and that sort of thing. I had come with the belief that we would find out about (the structure of) DNA by looking at its interaction with certain dyes, (a theme) which reappeared later in my life in another form. And I had some ideas about how DNA might make protein. I knew all the kind of classical genetics, or classical genetics that existed at the time, because going into microbial genetics made me read a lot of papers on what was then called biochemical genetics. And then, of course, in April of the following year, the three of us went on to Cambridge, and that’s when I met Jim (Watson) and Francis (Crick) and the model for the first time. And from that point on, that was it — I mean I knew what I was going to do (for the rest of my life)!
Friedberg: So, shortly after that you got to see what I would call “big science” in the United States, in about 1954?
Brenner: When I went there, it wasn’t big science yet. It was still pretty small science, at least for the subject that we were interested in. There were only a very handful of people (in this field then). In fact, there is a picture of the phage meeting of 1954 at Cold Spring Harbor, and the number of people is still tiny. Tens, not the hundreds or thousands that there would be (at such meetings) now. And I think the number of people who were then practicing the incipient science of molecular biology was still quite a small number. That science came from the fusion of genetics and biochemistry.
Of course, the big science in America at the time were biochemists, the departments of biochemistry. Big in the sense that they ruled the roost. That was the big part of biology being done at the time. But as you know, the big growth in American science took place in the late 1950s and early 1960s. And that was due to the launch of Sputnik by the Russians, which left the Americans feeling they were very far behind and therefore created a tremendous investment in science, which drew many more people into the subject; but it also came at the right time for us, because by that time, in the late 1950s there were scores — or more than a few, shall we say — of young people that had opted to go into the subject.
Friedberg: So when you finally left South Africa, why did you choose to return to the United Kingdom rather than the United States?
Brenner: I had decided that the place where I thought this sort of work would have complete momentum and where all the action would be, where things would have the right orientation, was Cambridge. So I returned to Cambridge, basically because there one had all the crystallography, that is the protein structure. This was one of the few places in the world where that was being done. The Americans had not yet got into protein structure. I had opportunities to go there, but I just felt that I would take my chances and go to England, because I liked the atmosphere of working in a place like Cambridge.
Friedberg: Tell us something about the 20-odd years that you shared an office with Francis Crick.
Brenner: When I came to Cambridge in the late 1950s, we had one room in which we had seven desks, where we also did our washing up of Petri dishes. And in fact Francis had his desk there. A number of people had their desks there, and I had a desk there. So we had very small lab resources. But I’m happy to say we got things going, and shortly after that we inherited a hut. And that hut was just outside the Cavendish Laboratory, and Francis and I moved into a joint office there, which we shared, and my lab was in yet another building. At one time, we were working in seven different buildings. And when we finally moved to the beginnings of the new site that is the present place of the Laboratory of Molecular Biology, we automatically moved into a common office, and we shared that until 1977, also when Francis went to America.
When we moved, I actually rescued the blackboards we had in there, and I rescued my desk. We had these blackboards on which we would write all sorts of things. In fact, there was a description of them by John Platt years ago in Science, I believe — I don’t think I could lay my hands on it now very easily, but it exists — in which he described that office and what went on in there, and why that sort of interaction was conducive to the generation of ideas.
One of the things was, we had an unwritten rule you could say what you liked, because I often found at least that when one first utters a thought, there is something wrong with it. It is only by discussing it and summing it up that, at least for me, I got onto the right track. I think it was mutual in many cases. Although there were other people like John Griffith, for example, who preferred to go away and work it all out and come back with a full answer. So I think he was more like a mathematician. I do believe that that was really conducive to generating all of these ideas and was a very good partnership. Because I’ve just been reflecting, and I think there’s only one paper that Francis and I wrote together as co-authors. And there are a few other papers, in which we both appeared (as co-authors with others), because we pursued different lines of the work, really. Francis was much more a heavy theoretician, and I liked and still do like the combination of a thought and action in the laboratory.
Part 3: Most Rewarding Scientific Contribution
At Cambridge, Brenner cites the experiments leading to analyzing the triplet code most intellectually gratifying.
Friedberg: What do you consider, Sydney, to be the single most rewarding scientific contribution in your life — in that period?
Brenner: I think the most rewarding thing intellectually was in fact the experiments leading to analyzing the triplet code. I think that intellectually was the most rewarding thing. And the reason for that is that it’s still remarkable to me to think that initiating that program, where one proposed essentially to try to find the genetic code by correlating fine structure genetics with the chemistry of a protein, was doable. But in fact to try to characterize DNA by genetics and recombination — that was a program which never got totally completed. The only part that got completed was the experiments that we did in Cambridge on, first of all, the triplet code and on interpreting the mutations there, and also another set of experiments, which were completed a little later on, working out which codons were which simply by mutagenesis. That’s the nonsense codons story.
So that I think was intellectually very rewarding, and I still think it’s an incredible experience that you could tell molecular structure, you could tell things about molecular structure, from just looking at holes in lawns of bacteria growing in Petri dishes. Whereas, as you know today, people do genetics by buying hundreds of machines and sequencing the DNA.
Friedberg: What about the discovery of messenger RNA?
Brenner: That, I think, had two features. One was, it was just the moment of sudden insight that all these observations were connected. I think everybody’s written about that time in my rooms (at Cambridge). That was a great thrill, just to suddenly realize that this Astrachan-Volkin RNA could be the messenger. Plus, of course, the big challenge of doing those experiments, which we did at Pasadena — and that of course was a huge challenge. But in fact the experiments were done and effectively proved the point. But talking about the intellectual thing—that was an insight followed by an experimental demonstration, and the realization that, of course, we’d all been thinking about the wrong kind of RNA for a long time. And just that formulation came as a thrilling insight as well.
Friedberg: In retrospect, do you think it’s remarkable that the experiments that you did at Cal Tech worked the first time?
Brenner: They didn’t work the very first time; they worked about the third time. They only worked when we got hold of the right magnesium concentration. So it was very frustrating. We did many of them up to that point, and none of them worked, and we only had three weeks to do them in. I think that those experiments were pretty close to being on the edge of doability. They were quite complicated things. And of course they worked the third time, once we got the magnesium thing right. And many people said, “Oh, it’s going to take years of work, you’ll have do hundreds of experiments, to find the right conditions.” I think it is remarkable that the insight of just bumping up the magnesium — which I think Francois Jacob has described in his autobiography — was correct. And in fact, when we had that insight, we ran back to the lab and did the experiment in the only time we had left to do it, and it worked just in time.
Friedberg: How did you come up with that particular experiment as your attempt to prove the existence of messenger RNA?
Brenner: We formulated (the experiment) very soon after the insight, and that’s the reason that we went to Cal Tech. There we would do a heavy/light transfer because we could separate the two classes (of ribosomes). In other words, we could separate old ribosomes from new ribosomes simply by this method of density gradient centrifugation. Of course, the famous separation (by density gradients) was the Meselson-Stahl experiment, in which they separated old from new strands of DNA simply by altering the density. And you can just see that that comes to the same thing. It’s the same logic that they used — but we were going to use it on ribosomes, arguing that if we could show that there were no new ribosomes made and that all the new RNA went onto old ribosomes, that’s all you’d have to do.
We also had to later prove that all the new proteins were synthesized on those ribosomes, because somebody could have argued that new RNA was just binding to inhibit the old ribosomes from making protein. It’s actually quite remarkable how many possibilities that had to be considered at that time now seem ludicrous. But one had to show that there weren’t a small number of ribosomes capable of protein synthesis. One had to show, and couldn’t show, that this was (actually) the messenger, because it could have been an inhibitor. It could have been something that switched off the old ribosomes. That’s all we could do then. But of course it was a peculiar base composition of the RNA, and that made the argument that it had been made on phage templates.
Friedberg: Are there any failures that stand out in your mind or favorite hypotheses that didn’t pan out?
Brenner: We had a lot of problems at the time in defining nonsense. In the present form, of course, everything corresponds to something. It either corresponds to an amino acid or it corresponds to chain termination. Now we didn’t know that at the time, so we could conceive of another kind of nonsense where you just get stuck. Suppose you had a configuration that could synthesize protein up to a point and then got stuck?
So we made a prediction that if we could damage the RNA in some way, we could effectively kill all the ribosomes. We tried to do this with X-rays. And what we expected here is that we would kill the ribosomes because they would load bad RNA, and it would get stuck and these ribosomes would be taken out of the system. Of course, that experiment didn’t work. But it’s very hard to think of a pet theory that we had to eliminate. The only thing that I think we held onto for a long time and tried to find is that we thought there might be a transfer RNA to recognize chain termination. Everything (we theorized) said it was quite possible; there would be a transfer RNA that recognized the three triplets, and instead of donating another amino acid to the end of the chain, it would essentially donate water and hydrolyzed it. I had some ideas about how this might be done based on some other biochemistry. So we did look for this RNA, and we looked long and hard for it, and of course we didn’t find it.
That was one example, but you know these aren’t things that demolish the entire structure of what you’ve been thinking about. I think we were nearly always completely on the right track. There were a few situations where we learned you just accept that that’s the way it is and you can’t to force it into anything else, and you give up the search. There were, of course, a lot of things that were very puzzling and which could not be fully explained until later.
Part 4: Transition from Prokaryotes to Higher Organisms
After the DNA structure was accepted, a period some scientists describe as an experience of anticlimax, it was exciting for Brenner as he turned to the study of “real living organisms.”
Friedberg: So Sydney, when the fundamental cornerstones of molecular biology were finally laid down, people like Francois Jacob and Jacques Monod, perhaps yourself and others, wrote about a huge experience of anticlimax. Gunther Stent did. He related that it was essentially all over now, and we might as well go fishing. So tell us something about how that period was for you, and your transition from prokaryotes to higher organisms.
Brenner: After 1961, around the time the code was solved and the DNA structure was fully accepted, we understood all about genetics at that level. In principal you could determine the three-dimensional structure of proteins, and you could sit down and say, “Well, what is one going to do now?” And you could say, “Well let’s try” — and this was one option — “to try and bring this knowledge to bear on the details of all these fundamental structures in the cell.” You could sit down and do a project, a molecular biology project, on ribosomes, for example.
We thought that basically we knew how phage was assembled. We did a lot of work to show that phages were assembled in bits and pieces, and that you could analyze these functions as well. So it seemed to me at the time that I needed to get out of all these tiny organisms and see whether this new approach could be directed at the old problems that I had started with; an interest in what was essentially genetics and the development of higher organisms. For me that was the direction I wanted to go, and I’d had an interest in the nervous system for a long time. I could now see that it was quite possible to adopt a genetic approach if one found the right organism.
Friedberg: So this period wasn’t — or it was — an anticlimax for you?
Brenner: Oh no, it wasn’t an anticlimax. I mean, the fact is that it would have been an anticlimax if one sat around and moaned all the time. But that was just saying, “Well let’s go and do something else.” And my only irritation with that time was that it took me a long time to leave the molecular problems behind. I was always drawn back into them in order to push them forward in the lab — like looking at all the mutations of transfer RNA at the time and showing which were the functional parts and so on. However, by about 1965, I managed to free myself from that and started this serious work on the nematode. And in fact, much of the things I wanted to do were the sorts of things I had done in South Africa, where I had been trained as an anatomist and histologist.
So it was quite fun getting back to that level again and seeing real organisms, real living organisms, and that still is the great thing about C. elegans. That’s how I got started back again, and although I dabbled for a couple of years with a few other organisms, eventually one settled on this one theme: using the technology of electron microscopy, which we realized would have to be done. Years ago, in South Africa, I had learned to serial section embryos and brains and do 3-D reconstructions of organs, and it just seemed that that was the technique to use to do this for a whole organism — but at the electron microscope level, where we could tell unequivocally where one cell began and another one ended.
Friedberg: So what was the original goal with C. elegans?
Brenner: The original goal of C. elegans was the following: to use genetic analysis to uncover the molecular basis, if you like, of the structure and function of a higher organism that displayed in essence all the features of arising from a single cell, differentiating into many different cells and having many cells of different functions acting coherently. The idea was simply to bring those tools that we had experience with. So the requirement was that we had to have an organism on which we could do extensive genetics. You also had to have a very powerful way of analyzing the mutants.
It seemed to me then that what we had to do was to analyze the cellular structure and function of this animal. So that was the rationale. And, in fact, right in the very first, the manifesto, we said we’re going to reconstruct the entire nervous system of this animal, and we’re going to find out where everything comes from in development. It was descriptive in the sense that we were just going to find out what was there, but we were going to do experiments. The experiments were going to be genetic experiments.
Now, of course, nobody had any idea then how genes would map onto the structure of complex organisms, how gene space would be mapped onto it. Often when I gave lectures in those days, people used to come to me and say, “Is there going to be one gene/one synapse?” I mean, was there going to be one gene/one cell? Nobody had any idea how these things would map. In other words, nobody had any idea what was the basis of gene regulation in higher organisms. Not the molecular basis, but the logical basis. So all we said, “Well we’ll just make mutants and study them as deeply as we can.”
But quite early on, we wanted to get some kind of molecular handle onto this. So we started very early to look at — because we found them — genes that affected the structure of muscle. We used to say myosin is going to be the beta-galactosidase of higher organisms, because there’s lots of it, you can purify it, and we knew a lot about its biochemistry, simply because of all the work done, the people who had studied molecular function of muscles. And so that seemed to be a good place to launch the kind of biochemical approach, in addition to the more anatomical approach. Then of course with the advent of cloning in 1975, we immediately saw that this could be brought to bear on this whole field.
Part 5: The Politics of Genetic Engineering
Brenner writes a paper for the Ashby Commission to dissuade legislation that he felt could inhibit scientific investigation.
Friedberg: Speaking of cloning, you witnessed the emergence of the recombinant DNA era, and in fact were very much involved in the early politics of this field. Can you elaborate on that for us a little?
Brenner: I actually tried to do a DNA recombinant experiment with C. elegans. And let me remind you that of course we had done a lot of genetic engineering by what we called “steam engineering” methods. We had captured genes onto bacteriophages by making specialized transducing phages. Of course, these were extremely rare events. And we had got to the stage of saying we would look at 10 to the 12 power events…. If we didn’t find it a recombinant in 10 to the 12 phages, we would know we would have to give up. So 10 to the 12 power events, we thought we could easily detect by selective methods. Lots of attempts were, and there were other people that I’ve talked to of trying to force DNA into bacteria and select for recombinants, of course, without much success.
Bacillus subtilis was readily transformable, and my argument was — it was completely naive and would never have worked of course for reasons that will be obvious in a moment — that we knew that lots of the enzymes of carbohydrate metabolism were the same, and that whether you took lobster or you took yeast or you took human trisphosphate isomerase, for example, they were essentially the same protein. And that we knew from protein chemists. So we thought that well, if we made a mutant defective in a carbohydrate enzyme in Bacillus subtilis, we could take C. elegans DNA and just put a hell of a lot of it into Bacillus subtilis and look for that rare recombinant where we might have complemented that mutation — and we were prepared to look at 10 to the power of 12 bacteria. Of course Francis Crick said I was crazy, it would never work. He was dead right. You have to realize we didn’t’ know about introns at the time. So even if the right gene did get in and even if it did recombine, it couldn’t have expressed.
Now, I was toying with doing this and Paul Berg came around to the lab and said he had this experiment where he thought he could the beta gal gene into SV40 and so on. I told him about my experiment, and said that I was still going to try it. Later I learned that other people had thought of doing almost analogous experiments, but never got around to it. But the next thing I heard about this was that there’d been this meeting at the Gordon Conference, where an announcement had been made that here’s this new method, and it could have its dangers and so on. And in fact there was going to be a moratorium on it. What happened immediately in England, we got a note from our boss at the MRC that we must desist from doing these genetic engineering experiments. And of course this was the good old time where again you saw the difference between impotence and chastity. Of course we didn’t know how to do these experiments. We didn’t have the resources to do them. So of course we didn’t do them, not because we had to stop doing them, but because we never started doing them.
And then they set up — very quickly to allay the fears of the public — something called the Ashby Commission. I wrote a paper for the Ashby Commission because I could already see that if this gets moving it’s going to create a lot of difficulty. I had to realize that in England this thing was taken very seriously because there had been an escape of smallpox from a lab in which quite a number of people died and hundreds of thousands of people had to be immunized. It was really a public health threat, because more and more people were not being vaccinated against smallpox; it could have had very dire effects. And against that background, this was being played out, in addition to the actual committees dealing with dangerous pathogens. Dangerous pathogens and the conjecturally dangerous bacteria were somehow linked together. So I wrote this paper for the Ashby Commission in which I pointed out several things. In fact what we got from this was that we should proceed with caution, do everything on a case-by-case basis, set up the right machinery, the typical English way of doing it.
So then the meeting at Asilomar took place, and Paul Berg — who knew of my interest in this and to whom I had sent a copy of my Ashby Paper — asked me to come to the meeting. Incidentally in my paper, I asked the following question: If I synthesized insulin, since it doesn’t come from an organism, how should we treat that? Because that’s just a chemical, it’s just chemical DNA? Now of course at that time synthesizing DNA was a little conjectural, but one knew that at the end of the day people would be able to synthesize genes. So what I was saying was that they had better get their thoughts right about this all this.
Part 6: The Politics of Genetic Engineering Continues
Brenner works in Britain to prevent moratorium legislation based on “fallacious biological theories.”
Paul Berg asked me to join the Asilomar Committee. I went across to Asilomar. I don’t know whether it has been generally recognized, but in fact the press were there. It was run by the National Academy, but the press were there in very large numbers. You have to remember that this is just after essentially the press had essentially gotten rid of a president, namely Nixon post-Watergate. They were really full of themselves. And in fact, on the first day there was a question that if someone didn’t want to be recorded by the Academy, which was making a record of the meetings, could he make his remarks off the record. In fact that was allowed so that people who didn’t want it noted what they said at the time could do this.
But the question was, should the press switch off their recorders as well? I have to say that when the vote was taken, I was the only one who voted that the press should switch off their recorders. At the press conference, which I had to take that evening, the reporter from the Washington Post just laid hell into me. He said, “You know this is a different country. You know, we have these things, and how dare you try to censure the press.” So I said, “Look I just believe in the right of all consenting adult scientists to make fools of themselves in private.” That was my remark. Anyway I got on pretty well with the man afterwards. But the interesting thing was, to me, that there was this thing that the press should make everything open. The public should participate. They want all the movements, all the science for the people.
So this whole thing took off on that basis — that there was a moratorium, that no one knew how you would escape from the moratorium. It is always a very bad thing to create a moratorium, without prescribing in detail under what conditions, what new knowledge, you would need in order to say, no, we don’t have one anymore. That was why after Asilomar, I realized the number one thing would be to get rid of the moratorium. That is why I sprang up, when I could see what was happening, and called for a vote. It was unanimous to stop the moratorium. Then we had to go with the other things.
Well, we had conceived of this idea of biological containment, because I’d been thinking about that from the Ashby thing. In fact the whole idea — one of my notes in the Ashby thing is on biological containment — that you could make bacteria, which would render genetic alterations harmless. It would just be part of the tools, so to speak, of genetic engineering. That you would have microorganisms that were confined to the lab, so to speak, and even if they escaped, they would be eliminated very quickly. Of course we’d also had this big experience in England which was antibiotic use in animal feed and the rise of antibiotic resistance, and that has to tell you it’s a public health nightmare. Now everything, all pathogens, are multi-resistant to multiple antibiotics, but that wasn’t because of genetic engineering. That was because of man’s intervention by environmental means, which have all been more severe than tinkering.
So one of the thoughts that we also had to get over was that — and it’s a thought that is still around a lot—is that certain genetic configurations have not been explored in the course of evolution. The one I used to like was the cloning of orange DNA in ducks. That combination has been only explored at the phenotypic level, but not at the genotypic level. Since we don’t know what the consequences are, therefore, we could create something that we don’t know about. Of course, what one has to do is to try to understand that these things are not unique, they are members of classes and in fact certain things almost like this have been explored in nature. So one had to say that nature is in fact the genetic engineer. Of course this is now accepted by everybody — it’s called horizontal transfer — and everybody just believes this now.
However, to go back there, there was something abnormal about moving DNA into organisms. And what happened thereafter was the NIH set up a committee to classify experiments and the conditions whereby they could be done, and there would be recombinant DNA safety committees and so on. I was on a similar commission in England, and we were very anxious not to install in any legislation, fallacious biological theories. You know one of the greatest fallacious biological theories that has been installed in legislation is that frogs don’t feel any pain because they’re cold blooded. So as you know, when the anti-vivisection laws were promulgated, only warm-blooded animals were considered. That’s neither here nor there, but it is a fallacious theory. I was very keen we shouldn’t do this, and we tried, although there was great pressure for us to be consistent with the Americans. Of course in America, it’s quite easy to change such things. In Britain, we tried not to create new laws or new regulations, but we subsumed this under some preexisting thing called the Health and Safety Executive.
But in Europe, parts of Europe, and especially in Japan, they copied the American system. And in fact they were stuck with it for 25 years because they couldn’t withdraw from it, because, of course, people correctly said, “What do you know now that you didn’t know five years ago that allows us now to change it? And you could answer that we have a different attitude, and of course, why did you put it in in the first place? It was insisted that our conditions should be very similar to those of the Americans, because the Americans were concerned about offshore genetic engineering. And if the English conditions were lower, then all Americans would come to Britain and do their experiments in UK labs, you see. So we had to have the same kind of level. But of course Americans began to change the conditions quite soon thereafter, and of course we had to have them changed in Britain. And it’s been quite a lot of one’s time doing this. But finally by writing a new scheme, which actually forced people to think quantitatively and logically and emotionally, we managed to get people to see that there was a rational basis for, say, that all it needed was good microbiological practice. And I’m happy to say that made a lot of work possible that would have just been very difficult. I consider it time well spent.
Sorry to give you this long story.
Friedberg: Very good. Sydney, thank you very much. Once again, congratulations.