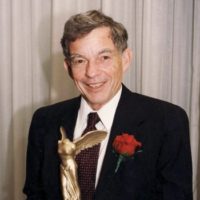
Clay M. Armstrong
University of Pennsylvania
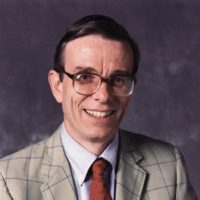
Bertil Hille
University of Washington, Seattle
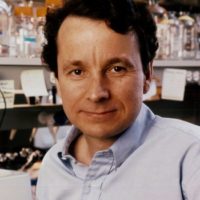
Roderick MacKinnon
The Rockefeller University
For elucidating the functional and structural architecture of ion channel proteins, which govern the electrical potential of membranes throughout nature, thereby generating nerve impulses, and controlling muscle contraction, cardiac rhythm, and hormone secretion.
Biologically speaking, human beings are frequently described in a number of ways. We are flesh and bone. We are comprised of essential life-giving organs neatly arranged beneath a sheath of skin—itself an organ of astounding complexity. We are made up of cells, genes, DNA.
It is less common to think of humans as electrical beings, but the description is equally apt. Like any good machine, humans are controlled by electricity—bodies made up of a vast network of interactive electrical components that surely surpass in intricacy those of any supercomputer.
This year’s winners of the Albert Lasker Award in Basic Medical Research bring to their work a unique appreciation of biology and a keen understanding of electricity.
Clay Armstrong is honored for his work in “cell membrane excitability” and the elucidation of “ion channel gating kinetics.” Armstrong himself puts it more simply: “My research involves electricity. Electrical signals are used throughout the nervous system and activate muscle cells. Essentially, we studied the electrical system that underlies all thinking and movement.”
As a medical student at Washington University in the late 1950s, Armstrong was captivated by lectures on the physiology of nerve impulses and by the work of A.L. Hodgkin and A.F. Huxley, who did pioneering studies of the squid, an elegant model for analysis of the passage of nerve impulses through cell membranes. Hodgkin and Huxley showed that the action of nerve cells is dependent on electrical conductance changes in the cell membrane. But the physical structures underlying these changes remained mysterious.
That is where Armstrong took the field to its next level, extending the Hodgkin-Huxley hypothesis as he himself adopted the squid as his experimental model for biophysical studies of the electrical system. Throughout, he always kept in mind the potential application to medicine of lessons learned from squid. “If all nervous system cells operate electrically, then determining the electrical mechanism has to be important,” he thought. Ion channels were the first step.
In order to understand the significance of the work of Armstrong and his co-winners, Hille and MacKinnon, it is important to understand ion channels as the basic component of the body’s electrical system. It is a concept that many people find difficult to grasp. Again, Armstrong makes it simple. “Ion channels are little holes in the membrane of all cells. The channels open and close to either permit or block certain ions from crossing the membrane. Sodium, potassium, calcium, and chloride channels are among the most important molecules in the electrical signaling system.”
Between 1964 and 1971, Armstrong developed evidence for gates that control the movement of ions into or out of a cell. He envisioned the ion channel as a long, thin pore, flanked by slightly wider “vestibules.” The narrow part of the pore determined its ion selectivity. Ions coming from one end could dislodge blockers that had entered the channel from the other end, providing clear evidence for the channel idea.
Hille describes Armstrong’s accomplishment this way: “He proved for the first time that a drug may block an ion channel by physically plugging the pore.” In some cases, an ion blocks the pore from the inside, as if “swept into the channel by the electrical field.” Alternatively, external “in-rushing” potassium ions can clear the “plug,” or block, from the channel in the membrane. In all cases, a drug can neither get into nor out of a cell unless the potassium channel gates are open. The study of potassium channel-blockers gave a surprisingly detailed picture of channel structure.
Throughout the 1970s, Armstrong and his team proposed what is called the “ball and chain” model of channel inactivation, demonstrating that the activation and inactivation of ion channels involves two separate physical structures. An “activation” gate covers the inner end of the channel. When this gate swings open after a change in electrical voltage, the channel conducts transiently and then a tethered peptide “ball” swings into place to block or inactivate the channel. The ball is held in place by a chain that can be severed by enzymes, leaving the activation gate unaltered.
Armstrong’s simple mechanical picture of a complex electrical system drew considerable skepticism in the beginning, but now is generally accepted.
Finally, Armstrong suggested a powerful explanation of the electrical or voltage dependent changes that precede the actual opening of an ion channel when he showed that the channel’s activation gate itself is comprised of one positively charged and one negatively charged helix—in short, a zipper.
Now Armstrong is focusing his energy on the idea that proteins in solution are key to understanding the phenomenon of ion channel gates at an even more basic level. And once again his notions are being met with doubt by some of his colleagues.
But as Hille says, “Clay has intuitions that seem to go well beyond the experiments and that much later seem to be right on.”
Bertil Hille, who shared Clay Armstrong’s belief in ion channels long before the proof was in, says they envisioned “holes in membranes” in the early 1960s and were convinced that the “holes have gates that open and close. But we were working on a black box, with none of the equipment that exists today. We believed that ions have to go through the right size hole to get into a cell, so the holes or channels must come in different sizes. And we wanted to know whether the gates that control ion flow are on the inside or outside of channels.”
Hille’s interest in the field took hold while he was a graduate student at The Rockefeller University, from which he received his PhD in 1967. It was Hille, working with nerve axons from frogs, who showed that channels are independent physical entities in the membrane, each site generating electrical signals that make it possible for cells to talk to one another. Selective block by several neurotoxins proved that there are discrete and separate channels for sodium ions and for potassium ions. Prior to Hille’s discoveries, many scientists assumed that ions could flow across a membrane at any point.
In addition to identifying specific channels in cell membranes, Hille was fascinated to know how a molecular pore could recognize the difference between the tiny sodium and potassium ions. He demonstrated that the channels are sized to accept one kind of ion or another. Their pores have the capacity to act as a molecular sieve. He established that the pores contain water molecules, which also contribute to the ion selectivity.
Hille’s description of ion flow in sodium channels explained how only one sodium ion can penetrate or permeate a channel at a time, shedding water molecules as it passes through a series of energy states before getting to the inside of a cell. In contrast, his description of potassium channels explained many of their known properties by assuming that there are several potassium ions in that pore at once.
In 1977, Hille, always keeping an eye on the medical implications of his research, described the molecular interaction of local anesthetics acting on sodium channels, thereby laying the foundation for understanding the mechanisms of anesthesia. “We discovered that many agents, including local anesthetics, are channel-blocking agents. They wait until the door is open on the inside of a sodium channel, then enter and sit in the pore so no sodium can enter the cell. Therefore, no electrical signal gets to the brain and pain is blocked. This picture borrows much from Clay Armstrong’s potassium channel work of ten years earlier.”
Hille’s study of these blocking agents also contributed to pharmacological understanding of the action of arrhythmic drugs on the heart.
Now, Hille’s research is directed to the role of ion channels in a variety of cell systems, particularly G-protein signaling and the control of neurotransmitters such as adrenaline, acetylcholine, serotonin, and dopamine. “We originally thought that only nerve cells had ion channels. Then we added muscle cells, and now we know that every cell has ion channels to make signals.”
Hille notes that everything from sperm to white blood cells to endocrine glands needs ion channels. Furthermore, he adds, “the number of kinds of known channels has grown as well. A single excitable membrane may contain five to ten kinds and our genome codes for more than 100.”
Hille is not only an original thinker; he is also a remarkable author and teacher. Virtually all of his admirers mention Ionic Channels in Excitable Membranes, a classic text first published in 1984. It is considered the scholarly bible of ion channels and is one of the more cited publications in the scientific literature. Hille currently is preparing the third edition.
Roderick MacKinnon is honored for his elucidation of the structure and function of potassium channels. His work provided the first molecular description of an ion selective channel.
MacKinnon’s achievement is described in this way by a colleague: “In only a decade, Rod MacKinnon has taught us how a potassium ion channel is built and how it can unerringly pluck potassium ions from a sea of sodium ions and conduct them [through the channel] at a rate approaching the diffusional limit.” And, through his work in crystallography, he uncovered “a structure of breathtaking beauty that reveals how evolution satisfied the apparently paradoxical requirements for high selectivity and high throughput. MacKinnon has forever changed the way we view all ion channels.”
MacKinnon graduated from Tufts University School of Medicine in 1982, but four years later he abandoned plans to practice medicine in order to pursue postdoctoral studies at Brandeis University, in the laboratory of his undergraduate mentor, Christopher Miller. “My scientific career in effect began at the age of thirty,” he says.
In Miller’s laboratory, MacKinnon began working on biophysical aspects of channel function. “I focused on the protein selective for potassium ions, because it happened to be the target of our lab.” In the past 13 years, MacKinnon has sought the answers to two compelling questions: What do potassium channels look like? And how do they work?
MacKinnon, well known for his penetrating analyses of ion channel function, adopted a formidable array of techniques to answer these questions. Using electrical measurements, MacKinnon deduced that a scorpion toxin blocks a potassium ion channel’s pathway. He then exploited the toxin to analyze the subunit structure, moving gates, and ion conduction pathway of potassium channels.
MacKinnon and his biophysics team combined electrophysiology and molecular biology to identify the potassium channel pore loop. Through a series of elegant experiments, they showed that the pore loop defines the essence of a potassium channel by forming its selectivity filter, conferring the ability to accept potassium and exclude sodium. The central feature of the pore loop is the “signature sequence,” found in all potassium channels from animals to plants to bacteria.
Little more than three years ago, MacKinnon made the boldest of his career decisions. He chose to extend his conclusions based on electrophysiology and molecular biology by focusing most of his effort on obtaining the crystal structure of a potassium channel—considered by many the Holy Grail of ion channel biophysics.
His research team at The Rockefeller University focused on the KcsA channel, a potassium channel with two membrane-spanning segments and the signature sequence. They crystallized the KcsA potassium channel and solved its structure at 3.2 Å resolution, sufficient to answer the classic questions raised in the Hodgkin-Huxley age of membrane biophysics: What is the physical nature of an ion channel? What are the determinants of ion conduction and selectivity?
The channel, as MacKinnon had deduced earlier, is a tetramer of symmetrically arranged sub-units. Four inner helices are arranged like the poles of an inverted teepee with the wide end near the outer side of the membrane. The pore loop amino acids form the selectivity filter in the teepee’s wide end, where potassium ions on their journey across the membrane interact with backbone atoms from the signature sequence amino acids.
Most impressive was the stunning visualization of three ions within the pore—two in the selectivity filter and one in a cavity near the membrane center. “Years of pharmacology and ion permeability studies were suddenly understandable at a new and deeper level,” says MacKinnon.
Although the potassium channel can be elegantly described in the language of molecular biology, MacKinnon (like Armstrong and Hille) has the gift to put things simply. “Biological systems have small components that enable them to produce electrical activity. They are not entirely unlike toasters or televisions that have resistors, capacitors, and various other little parts. The potassium channel is one of the basic components or pieces of hardware in the body that carries ions to create electrical activity.” Of course, electrical activity is essential to life.
Armstrong and Hille were thrilled by MacKinnon’s paper. “It was the very first time we actually saw an ion channel,” says Hille. MacKinnon proved beyond doubt that many of the original hypotheses of Armstrong and Hille were right. Clay Armstrong calls MacKinnon’s structure “a dream come true for biophysicists.”
As is characteristic of great scientists, Armstrong, Hille, and MacKinnon are pursuing ion channel studies to achieve a more thorough comprehension of how the living molecules are built and how they work.
Key Publications by Clay Armstrong
Armstrong, C.M. (1971) Interaction of tetraethylammonium ion derivatives with the potassium channels of giant axons. J. Gen. Physiol. 58: 413–417.
Armstrong, C.M., Bezanilla, F., Horowicz, P. (1972) Twitches in the presence of ethylene glycol bis(-aminoethyl ether)-N, N’-tetraacetic acid. Biochim. Biophys. Acta 267: 605–608.
Armstrong, C.M., Bezanilla, F., Rojas, E. (1973) Destruction of sodium conductance in inactivation in squid axons perfused with pronase. J. Gen. Physiol. 62: 375–391.
Armstrong, C.M., Bezanilla, F. (1973) Currents related to the movement of the gating particles of the sodium channels. Nature 242: 459–461.
Armstrong, C.M., Bezanilla, F. (1977) Inactivation of the sodium channel. II. Gating current experiments. J. Gen. Physiol. 70: 567–590.
Armstrong, C.M. (1981) Sodium channels and gating currents. Physiol. Revs. 61: 644–683.
Key Publications by Bertil Hille
Hille, B. (1967) The selective inhibition of delayed potassium currents in nerve by tetraethylammonium ion. J. Gen. Physiol. 50: 1287–1302.
Hille, B. (1971) The permeability of the sodium channel to organic cations in myelinated nerve. J. Gen. Physiol. 58: 599–619.
Hille, B. (1977) Local anesthetics: Hydrophilic and hydrophobic pathways for the drug-receptor reaction. J. Gen. Physiol. 69: 497–515.
Dwyer, T.M., Adams, D.J., and Hille, B. (1980) The permeability of endplate channel to organic cations in frog muscle. J. Gen. Physiol. 75: 469–492.
Pfaffinger, P.J., Martin, J.M., Hunter, D.D., Nathanson, N.M., and Hille, B. (1985) GTP-binding proteins couple cardiac muscarinic receptors to a K channel. Nature 317: 536–538.
Herlitze, S., Garcia, D.E., Mackie, K., Hille, B., Scheuer, T., and Catterall, W.A. (1996) Modulation of Ca 2+ channels by G protein beta-gamma subunits. Nature 380: 258–262.
Key Publications by Roderick MacKinnon
MacKinnon, R. (1991) Determination of the subunit stoichiometry of a voltage-dependent potassium channel. Nature 350: 232–235.
Heginbotham, L., Abramson, T., MacKinnon, R. (1992) A functional connection between the pores of distantly related ion channels as revealed by mutant K+ channels. Science 258: 1152–1155.
Root, M.J. and MacKinnon, R. (1994) Two identical noninteracting sites in an ion channel revealed by proton transfer. Science 265: 1852–1856.
Hidalgo, P. and MacKinnon, R. (1995) Revealing the architecture of a K+ channel pore through mutant cycles with a peptide inhibitor. Science 268: 307–310.
Doyle, D.A., Cabral, J.M., Pfuetzner, R.A., Kuo, A., Gulbis, J.M., Cohen, S.L., Chait, B.T., MacKinnon, R. (1998) The structure of the potassium channel: molecular basis of K+ conduction and selectivity. Science 280: 69–77.
MacKinnon, R., Cohen, S.L., Kuo, A., Lee, A., Chait, B.T. (1998) Structural conservation in prokaryotic and eukaryotic potassium channels. Science 280: 106–109.
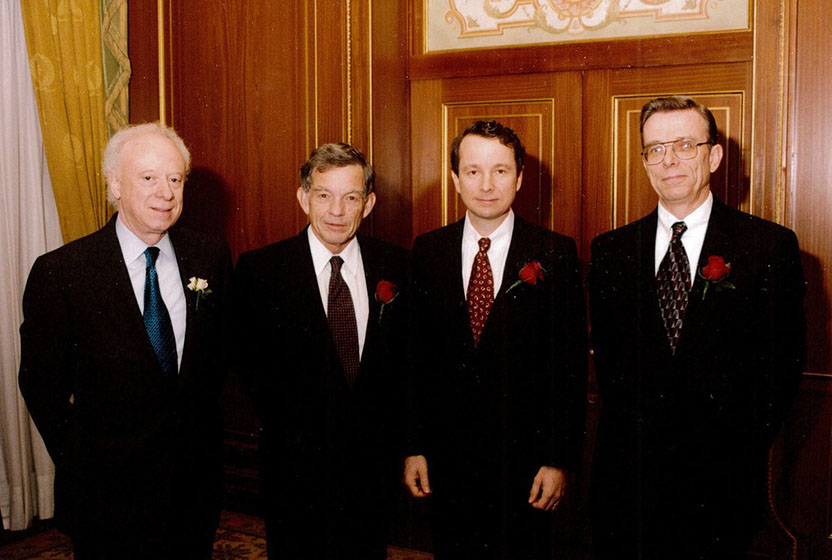
Joseph Goldstein, Clay Armstrong, Roderick MacKinnon, Bertil Hille
Award presentation by Denis Baylor
It’s a pleasure to introduce the winners of this year’s Albert Lasker Basic Medical Research Award: Clay Armstrong, Bertil Hille, and Roderick MacKinnon. Clay, Bertil and Rod have made outstanding discoveries about some of the most important molecules in living cells: ion channels. Ion channels are specialized protein molecules in cell membranes. They generate electrical signals in nerve cells and muscle fibers, produce the cardiac rhythm, and instruct glands to secrete. An individual channel molecule is an on/off switch that controls the flow of ions across the membrane. When switched on, it allows a specific type of ion—Na, K, or Cl—to cross the membrane. These ion movements produce small electrical currents that change the membrane voltage and transmit information from one place to another.
An ion channel is a remarkable molecular machine. Typically it sorts ions one at a time, yet allows the preferred species to pass through at a rate of a million per second, far higher than the speed at which the best enzymes can process their substrates. One of several types of signal can activate channels. Some switched on by the membrane voltage itself have a voltage sensitivity tenfold higher than that of the logic gates in a spanking new computer. Wonderful as they are, channels do not always work properly, and malfunctions produce an expanding list of diseases that includes epilepsy, cardiac arrythmias, and cystic fibrosis.
Before Clay, Bertil and Rod’s work, physiologists knew that ion channels are important, but what channels actually are and how they work were only understood at the level of the formalisms that Hodgkin and Huxley had enunciated two decades earlier. Hodgkin and Huxley’s work, which earned the Nobel Prize in 1963, had revealed that separate pathways allow ions of different types to cross the membrane, and that, for certain neuronal channels, the voltage across the membrane determines whether ions are allowed to pass or not. It was not clear how ions pass through channels, how channels achieve their remarkable selectivity for ions, nor how the voltage across the membrane instructs the channel to allow ion flow or to block it. The complete molecular structure of a channel? Forget it! This of course was before our awardees came on the scene.
Bertil Hille, from the University of Washington, provided a physical mechanism for ion selectivity. He did so by characterizing the gauntlet an ion must run as it traverses the channel’s pore. The approach was beautifully direct: find the size of the hole in a sodium channel by trying a variety of ions and determining the largest that can squeeze through. The bottleneck he measured would allow a sodium ion to pass only after it had shed all but a few of the water molecules that normally surround it. Loss of the waters was energetically compensated by negatively charged carboxyl oxygen atoms in the pore wall. This picture explained how a sodium channel effectively allows only sodium ions to pass, yet lets these “chosen” ions through at a very high rate. Bertil extended the analysis to potassium channels and showed that they achieve selectivity in a more subtle way, stripping potassium ions of water and allowing multiple ions to dwell in the pore at one time. Electrical repulsion among the ions allows them to traverse the pore at very high rates even though they interact strongly with the pore walls. More recently, Bertil discovered that some channels are regulated directly by interactions with G-proteins, ubiquitous signaling molecules of the cell interior. He continues distinguished investigations on fundamental mechanisms of cell signaling. Bertil’s book, Ionic Channels of Excitable Membranes, is prized by students and senior scientists alike for its depth and clarity.
Rod MacKinnon, at The Rockefeller University, did the unthinkable: He crystallized a potassium channel and determined its entire molecular structure by X-ray diffraction. Earlier he had used physiology and molecular biology to demonstrate that a potassium channel consists of four subunits and to identify the amino acids that line the channel’s outer vestibule and pore. The next logical step was to visualize the complete structure of a potassium channel. Doing so required him to test countless crystallization conditions, construct many mutants, and in his off hours learn X-ray crystallography. He did all these things in short order, finding a beautiful structure built from four subunits that come together to make an inverted teepee spanning the membrane. The form and makeup of the pore provide a firm structural basis for the high potassium selectivity and ion transport rates in these channels. Indeed, as Hille, Hodgkin and others had inferred, multiple potassium ions reside in the pore. Now the channel that Rod crystallized was from the bacterium Streptomyces lividans. In a beautiful sequel to the structural work, Rod showed that the bacterial channel can be blocked by a scorpion toxin that blocks potassium channels in higher organisms. This indicates that the structure of the potassium channel’s mouth was conserved as higher organisms evolved. Rod is extending this work to other channels, and we eagerly await his next structure.
In closing, it is clear that Clay, Bertil and Rod’s fundamental insights into these key molecules will become ever more important in understanding and conquering human disease.
Interview with Clay Armstrong and Denis Baylor
Denis Baylor, Professor, Department of Neurobiology, Stanford University, interviews Clay Armstrong, who shares the 1999 Albert Lasker Basic Medical Research Award with Bertil Hille and Roderick MacKinnon, November 1999. Dr. Armstrong is a professor of physiology at the University of Pennsylvania in Philadelphia.
Part 1: Ion Channels in Medicine and Biology
“I think that ion channels are the most important single class of proteins that exist in the human body or any body for that matter,” Dr. Armstrong tells a hypothetical skeptic from medical school.
Baylor: So, Clay, it’s really good to talk to you today.
Armstrong: Well, thank you, Denis, it’s mutual.
Baylor: Well, I have a tentative plan for this interview, so I’ll tell you in an outline what the tentative plan is. We can follow it more or less closely, depending on how it evolves. I wanted to ask you a little bit about how you see the place of ion channels in biology and medicine and then ask you a little bit about your family background and your family today. Then talk a little bit about your education and training in science—mainly the places and the people. And then really get into the meat of the science after that and talk about how you came to your wonderful insights into channel structure and function. And then talk a bit about what you’re up to currently. And then the future. And then what you see the future of ion channel work being and what advice you might have for young people that are considering a career in science. Would that be reasonable?
Armstrong: Okay. Yes. That sounds fine.
Baylor: That’s some outline of what we might do.
Armstrong: Wonderful.
Baylor: So, about the place of ion channels in biology and medicine. Suppose that a skeptical medical student came up to you and said, “Okay Dr. Armstrong I have too much to do. Why should I care about ion channels?” What would you say? (Laughter.)
Armstrong: Well, I’m confronted with that situation quite frequently. I’ll be aggressive about it—I think that ion channels are the most important single class of proteins that exist in the human body or any body for that matter. And of course, the question is something like saying, “Which is the most important part of a car, the tires or the carburator?” You can’t do without either. But all of the higher functions, all of the communication between the parts of the body, depend very crucially on the function of ion channels, and every perception, for example, is encoded in electrical form through the function of ion channels. Of course in your wonderful work on the retina and on the visual receptors, that’s certainly the case that the ion channels are involved in the initial transduction of light into electrical impulses, which are then dealt with by the brain. The heartbeat is another example. It is an electrical timing system dependent on ion channels that produces a heartbeat. All of our thoughts, all of our motions involve the action of millions of ion channels—billions. Endocrine secretion. All of these are, of course, are in addition to the more basic functions of ion channels, which are involved in the very life of the cell—of every cell. No cell could exist without ion channels. So I regard it as extremely important and also medically important.
Baylor: Definitely. And it seems that these ion channels now have an increasing significance in medicine, because a lot of neurological diseases turn out to involve mutations in ion channels, don’t they?
Armstrong: Yes, yes indeed. Well, epilepsy, for example, is one clear case. Myasthenia gravis is another. Various of the paralyses and myotonias. And that’s leaving out, of course, the big one—which is not neurologically related—but the function of the heart, which is certainly one of the most important medical problems to be encountered.
Baylor: Definitely. And so the channels also, I guess, are going to be very important, increasingly important drug targets for medical therapies of one kind and another.
Armstrong: Yes, well that certainly seems clear, Tranquilizers, of course, are one of the more (important categories)—I think no one really knows. With some of the tranquilizers it’s clear what the function is, but looking through the Merck index there are, oh, thousands of compounds that have been discovered empirically that do not have clear functions. And I would suppose that very large numbers of those must modify the action of ion channels in one way or another. Potassium channels are becoming clear targets of therapeutic intervention in, for example, diabetes and also in problems of arrhythmias of the heart. And again, going through the list of things that we don’t understand. Thought, for example. Well, nobody knows in any precise detail how thought occurs, but that thought involves the use of ion channels seems very clear since all communication between cells and all communication within cells depends on ion channels. Well, it’s just beginning. The only reason that the medical student could possibly think that it is not important is that we don’t know enough yet.
Baylor: Yes.
Armstrong: And also I think that it is not a very popular subject. So generations of preceding medical students have told the hypothetical students that I’ve just encountered (in your question) that you don’t really need to know that because it—in a sense it’s perfectly reasonable—there’s not enough that you can do about it at present. But that’s definitely not the case in the heart or in the case of diabetes.
Baylor: And it really seems a certainty that down the road these ion channels will be essential—that medical students must know them cold in order to be good physicians.
Armstrong: Well yes, and to be medical scientists. The medical schools are supposed to be training medical scientists who will take things forward. So (for) all of those students who are hoping to shape the future of medicine, I think this is definitely a place where one should look. The problem generally speaking is that medical students, I think, have very good minds. They have retentive minds, but they tend to be somewhat less analytical than the students who go into physics and other pursuits like that. So they are not at present well trained in some of the things that one needs to know in order to get over the hurdle of understanding ion channels.
Baylor: I agree. Well, I think that’s a pretty convincing answer. If the student doesn’t believe you now, then maybe he shouldn’t be in medical school.
Armstrong: Well, I would get read off of the faculty here if I said that too loudly, but I think one has to convince (not coerce).
Armstrong talks about his parents, his brother, his wife (who is also a scientist), their four children and his interests in marathons and mountain climbing.
Baylor: So Clay, could I ask you a little bit about your family background? Where you grew up and your folks and brothers and sisters and stuff like that?
Armstrong: Oh sure. Well, I came from the Armstrongs and the Margraves, and the Armstrongs and the Margraves converged on North Texas after the Civil War. And then both families, my maternal family, the Margraves, and the Armstrongs moved to Southeast Oklahoma. And oddly enough, it’s a corner of the world that I thought no one would ever know about, and I discovered that, in fact, Chris Miller had family connections in Hugo, Oklahoma, which is where my maternal grandparents grew up.
Baylor: I didn’t know that.
Armstrong: Yes. That was very interesting to hear about. But in any case, the Margraves, my grandfather and grandmother, had four daughters who were all lovely, interesting and intelligent. My father was the only surviving child of the Armstrongs, although my grandfather had 12 brothers, so that was a very large family. My grandmother (Armstrong) died at a relatively young age, so they had only two children, one of whom did not survive. My father went off to join the Army during the First World War and never finished college. I was born in Chicago, where he was working in a bank until the Depression really hit strongly, and then we moved back to, first Oklahoma and then finally to Dallas, Texas, where he worked in the Federal Reserve Bank. My maternal grandparents continued to live in Hugo, Oklahoma, and my paternal grandfather was a lawyer in a little town called Idabel, which was about 40 miles away. Well, it was an interesting place to visit, both of them—the cotton gins and so on. I have one brother and many, many cousins. It was always a very strong family because of the ties among the Margrave girls, who were really, really entertaining. My brother, in fact, lives in California, not too far from you. He lives in San Jose, and is a very entertaining and intelligent person, always interesting to talk to on virtually any subject.
Baylor: But you were the first one from your family then to go into medicine and science.
Armstrong: Yes. Of the two of us, Bob was more interested in history and English literature, and I went into medicine.
Baylor: And your family today is pretty interesting. I know your wife, Clara, is a distinguished scientist in her own right.
Armstrong: Yes, yes, well Clara is certainly more famous, deservedly, than I am.
Baylor: And do you two talk science? Do you talk to her about the structure of the release apparatus in muscle fibers, and does she talk to you about how channels work?
Armstrong: Yes! We talk to each other quite a bit. It’s usually not so much about specific questions of our own work, but just general knowledge about cell biology and things of that sort. I learned a very great deal from Clara, including a positive attitude that, uh—I tend to be somewhat skeptical. Clara, on the other hand, is always enthusiastic about new work. And so her lessons to me in that regard have been very helpful, because I think in general that one learns much faster if you accept a new proposal and then have a very enthusiastic attitude toward it, and then later perhaps you can become more critical about it. But the general initial acceptance I think is an important step in learning about it.
Baylor: Interesting, although the scepticism’s not all bad either.
Armstrong: No, no, but I think that should come a little bit later, after you have some grist for the mill.
Baylor: And you have several kids, right?
Armstrong: We have four children, John, a son, and three daughters. John works for Compaq computer. Katie is married and living in Palo Alto, as a matter of fact, and she teaches school and her husband is there at Stanford–Will (Talbot). Sandra plays the viola and is actually a professional, managing to make a living in what must be the hardest occupation of virtually any, because the competition is fierce.
Baylor: Definitely. That is very impressive.
Armstrong: But they actually heard a piece of very good news. They’ve been selected to play a new contemporary piece and will be paired on a CD with the Juillard Quartet. So that’s spectacular.
Baylor: Wonderful.
Armstrong: I’m extremely pleased to hear that.
Baylor: Congratulations. That is wonderful.
Armstrong: And Cecilia is—well, as you know, since she’s working with one of your colleagues—is a postdoc at the University of Washington with Fred, and well she’s a delight, as they all are.
Baylor: She’s really going to enjoy working with Fred Rieke. He’s just a terrific young scientist. And I know that you like to run in your spare time. You’ve been doing this for many, many years.
Armstrong: I guess it’s not quite correct to say that I like to run, but I do run, yes. I enjoy it part of the time. Running competitively is actually kind of fun, so I have even won a few races—in my age group.
Baylor: Wonderful. Have you done a marathon?
Armstrong: Oh, yeah. I have done six or eight marathons. I won my age group once upon a time in the Philadelphia marathon, so that was nice.
Baylor: Fantastic. You also do some mountain climbing with Clara I hear.
Armstrong: Oh yes, yes. Clara has turned out to be a very good mountain climber, so we had a wonderful trip to Nepal this spring, as a matter of fact. And we were walking around at very high altitudes for, oh, three weeks, I guess, in a place called Inner Dolpo, which was extremely remote. A very, very fascinating and trying trip. Both of us absolutely love that and would love to be back there even now. That’s our second trip to Nepal. We had one preceding trip in which we went to the Everest region and got up quite close to the foot of Everest.
Baylor: That must be an amazing sight.
Armstrong: Oh, it is spectacularly beautiful. I love the Sierras, where we’ve also walked with great fondness, but the angle of vision involved in taking in these mountains in Nepal and particularly in the Everest region is, well, one certainly has to crane one’s neck to see the tops of things. It’s just astounding to see how tall they are and how beautiful. Every corner has a new vista that is amazing to see.
Part 3: Education and Training
A graduate of Rice Institute in Dallas, Armstrong went to medical school at Washington University, where he first became interested in electrophysiology.
Baylor: Clay, could I ask you a little bit about your education and training in science? Mainly the places and people for the time being, and then we’ll get to the nitty gritty of TEA and such in just a little bit. But could you tell me just a little bit about your educational odyssey?
Armstrong: Okay. Well, life is a matter of chance. It didn’t start off that way, but there were many points where chance was a big factor. I went to Sunset High School in Dallas, Texas, and worked reasonably hard as a student. So I managed to get accepted to Rice Institute, which was certainly the best school, I would say still is the best school in Texas. I went there for my undergraduate degree, and I was very fond of the place—still am. It was very rigorous in those days and not, I think, quite as accommodating to the students as it has become since. I think a third of our freshman class didn’t return for the second year either because they hadn’t made it academically or just didn’t want to (return). But the education there was absolutely first rate. The teachers, the professors were all in love with their subject. And they managed to communicate that very effectively. So, I took a course that was relatively heavy in science and then proceeded to Washington University, which is and was a most excellent medical school. And I guess I had certain adjustments on leaving the ideal world of chemistry, physics, and coming to the much more empirical world involved in medicine.
Baylor: It is a different way of thinking, isn’t it?
Armstrong: It certainly is—and necessarily. I mean, one can’t be too theoretical about medicine, because it’s an empirical subject largely. So anyway, that was an emotional shock. And with any emotional shock, as I’m sure you’ve experienced, you feel that it’s improper, that you’ve learned the right things already, and anything that goes counter to either the feeling or the substance of what you already know must be improper. So certainly I had all of that feeling in trying to cope with medicine. But the first year, I was very fond of it. I liked biochemistry, even anatomy I liked a lot. But then in subsequent years when it came to pathology—for one thing I was color blind, so I couldn’t tell the pink from the green tissues. But it was there in Washington University that I first got interested in electrophysiology.
Baylor: Oh yes, that was the place of Gasser and Erlanger.
Armstrong: Yes, right. And George Bishop was one of their people (colleagues). Very strong minded, very intelligent, wonderful man, who was the electrophysiologist-in-residence of the Neurology Department at Washington University, and it was in his general environs that I was working as an electrophysiologist. In fact, I was in the screened room right next to his office and using equipment that he had designed for measuring brain waves. And that was quite a fascinating learning experience. I actually learned some things about the brain and the evoked potentials in the visual system that I think still would be worth investigating.
Baylor: So this was work on the connections between the lateral geniculate and the visual areas 17, 18 and 19?
Armstrong: Yes. Yes. I was there working in the era, at roughly the same time as Hubel and Wiesel were doing their wonderful work on the connections of, well, basically the wiring of the visual system and the various types of receptive fields in areas 17, 18 and 19, and then the lateral geniculate. Well, one of the questions (facts) that came out, that was kind of obvious from, not just my work, but from the preceding work that George Bishop had done in analyzing the evoked potentials in the same areas, was that there are direct connections from the lateral geniculate body to areas 18 and 19. And Hubel and Wiesel, I think, at least initially tended to think more in terms of serial projection from lateral geniculate to area 17 and then from 17 to 18 and so forth.
Baylor: That’s right. Certainly that was one of the big points or fairly big points of their work.
Armstrong: I think it is now clearly recognized by everyone that, in fact, there are direct projections from the lateral geniculate to some of the higher numbered areas. My favorite from the point of view of getting attractive looking evoked potentials was area 18. And there one could analyze the extra cellular field potentials and discover a synaptic potential in layer 3(b). One could see, in my analysis, firing of the initial segment of the axons projecting downward from the layer 3(b), firing of the cell body, and then activity that had to be in the apical dendrites of the pyramidal cells in that region. And then there was a quite fascinating story to my mind about inhibition. If you preceded a strong stimulus with a very small stimulus through the same electrodes so that this was all firing one family of axons projecting from the lateral geniculate to area 18, you could completely suppress the post synaptic activity in the layer 3(b) pyramids with this small preceding shock. And you had to allow an interval that was sufficient for transmission through one extra synapse. So it all fitted with the idea that this one family of axons had two connections. Direct connections that were excitatory to the layer 3(b) pyramids But the same family (of cells) then received inhibitory input such that one could imagine that those same axons coming from the geniculate synapsed with inhibitory cells (which) then inhibited the layer 3(b) pyramids. That was in general quite consistent with the picture of Hubel and Wiesel. One could easily synchronize this, harmonize this with their story on the inhibitory surround for the slit receptors that they found in area 17.
Baylor: It’s a very elegant result.
Armstrong: Well, I think it’s nice. I have always thought that I would like to return to work on that at some point. So anyway, that was my introduction to electrophysiology at Washington University. And following that I interned for a year at the University of Chicago and then came back for a short time to Washington University and was fortunate enough to find a place in Kacy Cole’s lab at the National Institutes of Health.
Part 4: Leaving Medicine for Scientific Research
Armstrong describes why he left medicine to join Kacy Cole’s lab at the NIH. Later, he and his wife work in the lab of Andrew Huxley in London. Next, he went on to Duke and then Rochester before arriving to stay at the University of Pennsylvania.
Baylor: Now was it a difficult decision to leave medicine and go into science, or was it easy?
Armstrong: For me it was not a difficult decision. I had decided that, again, my mind was just not the right sort for medicine. That I preferred dealing with things that were idealized and less empirical. I think also the general tenor of the discussions that one had on (medical) rounds was somewhat difficult for me, because it was all focused on showing that one had read the latest articles in the medical journals. So it struck me as somewhat too empirical, and I think that my inclination was to try to improve the basis for understanding some of these things. I might say that I keep watching these things, and there has been unbelievable progress in many fields. It really makes me proud to be a part of the medical science endeavor to see just how rapidly things are changing. Immunology is one of the specific cases that I can think of. Immunology sounded, when I first heard about it in medical school, like something that I didn’t want to know anything about. But now it is, after many years of effort, a wonderful story.
Baylor: Right. So you went back to Washington briefly and then went to NIH to Kacy Cole’s lab—the lab of biophysics. And that must have been very exciting.
Armstrong: Oh, that was absolutely wonderful. For one thing, it was, of course, a complete change of surroundings. I’d gone from Texas to the Far East, as I saw it, and of course everything was different. Washington was a wonderful place to be. The entire atmosphere there was extremely stimulating. So I felt very fortunate to be able to go to NIH. NIH at the time was really a wonderful center, which was very helpful in stimulating the development of medical research in the universities and medical schools. It played a very key part, and I happened to be there just at one of the very best times imaginable.
Baylor: And then from NIH it was to Andrew Huxley’s lab?
Armstrong: Yes. And that was also a wonderful learning experience. Huxley was really a very exceptional man. He was so brilliant, with such a powerful mind, and so imaginative in his experiments. The list of his accomplishments is really quite staggering.
Baylor: So you worked with him for about one year, was it?
Armstrong: Clara was also working in his lab, and we were there for two years. He was quite busy at the time as department chairman, so the experiments really didn’t quite get started, but as a learning experience it was really spectacular. The reason that the experiments didn’t get started, I might say, is that I had to learn how to dissect single muscle fibers, which is very hard. So it was my deficiency as a dissector. Although I had learned to dissect squid axons by that time, muscle fibers were something else. It was difficult to get a very good experiment out of the fibers that I could produce.
Baylor: So from UCL (University College London) you went to Duke and then to Rochester?
Armstrong: Yes. Duke at the time was a very important place in American physiology. Dan Tosteson was the chairman and Paul Horowitz was a huge attraction for me. And it was a wonderful department. Certainly one of the best that I’ve encountered. Again, a very stimulating time. John Moore was there working on ion channels, and so we loved it at Duke. It was a very good place to work. We then followed Paul Horowitz to Rochester and that again was a wonderful place to work. Paul’s department was splendid. So many friends from those institutions. And in the winters, one of my connections at the time from both Duke and Rochester was with Chile. I had first gone to Chile when I was at NIH in Kacy’s lab…
Baylor: In search of squid.
Armstrong: Yes, in search of squid. And there were two big inducements to go to Chile. One was that the atmosphere was very lively. They were a wonderful group. The Chileans have crazy work schedules. They work all the time and they’re very lively. So that was one big attraction. Then the other was that they had squid that were just very large so that it made the dissection of the axons and the performance of the experiments very easy. It was there in Chile that I first encountered Pancho, who is one of the best things that every happened to me.
Baylor: Wonderful colleague.
Armstrong: Yes. So then, well in brief, after approximately six years at Rochester we came to Penn and have been at Penn ever since. My connection now is not with Chile, but with the Marine Biological Laboratory (in Woods Hole, Massachusetts), which is where Clara and I met, as a matter of fact. I first went to MBL while working in Kacy’s lab at NIH, and Clara and I met there. Clara at the time was a postdoc with Keith Porter. She was at Harvard, so she came down for the summer, and we met, and it was love at first sight, I guess. (Laughter.)
Baylor: And MBL is certainly a wonderful place for science, isn’t it? It’s stimulating, and people from all over the world come and make do even if the equipment isn’t perfect. Somehow people manage to find some sealing wax and thread and make it work somehow.
Armstrong: Yes, well, that you have to do. The MBL is really one of the places of my dreams. It’s very supportive from the point of view of research. People work very hard, so the reward is there all around you. It’s such a beautiful place. I think that contrary to the impression that people go to play tennis and enjoy the beach, I think that although those things are there, it just makes people work all the harder. So I absolutely love the place.
Part 5: Ion Channels as Definite Physical Entities
Armstrong elaborates on his early work using TEA and his collaboration with Dr. Hille.
Baylor: So Clay, could we get into the nitty gritty of your work? I think that to an outsider, one of the most striking features of what you’ve done is to think about the channels as definite physical entities, as little machines that work according to certain rules that are mechanistic rather than these formal entities that had been conceived before you got into it. And so I would love to ask you about how this evolves. I guess TEA played a big part in a lot of the initial thinking about how channels work and the idea that you could flush TEA out of the channels with potassium moving in and so on?
Armstrong: Um, hum. I think the way that you put it is very accurate. When I first arrived in Kacy’s lab, I think that the emphasis was largely mathematical. Well, for example the ideas of pores and carriers somehow just weren’t on the map. One wanted a mathematical formalism to explain what we now call gating, and Kacy at the time was trying to understand it in terms of electrodiffusion of ions within the membrane according to the Nernst-Planck ideas and formulation. Being a practical mechanical type from Texas, I tended to want to know a little more of what the conducting units as they were called at the time looked like, and so I was very attracted by the pictures given by Hodgkin and Keynes of the long pore effect. That was in existence, of course, that was part of the educational background.
Baylor: That was a wonderful mechanical model that they had with the two big containers full of balls that were being shaken back and forth.
Armstrong: Yes. That was very stimulating. I tended to immediately think in those terms. And also I was, well, lucky to some degree, but I think kind of I would have to say thoughtfully lucky in getting involved with TEA. And that I did just by going to the library and reading. I read two papers that I found to be very stimulating. One was by Hagiwara and Tasaki, and they had done something which clearly looked to an unbiased observer like they were blocking potassium channels with TEA. And that’s what Hagiwara always said that he thought, but that he couldn’t say it because Tasaki was too strongly against the idea of ion channels. But in any case, that was one of the papers. And the other one that I read that I thought was just wonderful was a paper by Dennis Noble about anomalous—which subsequently became inward—rectification in the heart. I was very stimulated by that paper and decided on the basis of quantitative measurements given in the paper of Hagiwara and Tasaki that the potassium channels must be behaving as inward rectifiers. So well, then the attempt was on to prove it using squid axons during the summer, and it eventually worked out. Certainly it didn’t seem for quite some time, of course, that it was going to. But Leonard Binstock and I were performing experiments together, and we indeed discovered that if the potassium concentration was high on both sides and TEA was present on the inside, that the potassium channels behaved like inward rectifiers.
Baylor: That must have been very exciting.
Armstrong: Oh, that was terrific.
Baylor: You were immediately then thinking that the potassium moving inward was knocking the TEA out of the channel?
Armstrong: Well, I was trying to imagine what TEA could be doing, so I made a CPK model of TEA and discovered that basically it couldn’t be doing very much, because it’s kind of a little solid pyramidal chunk with its central nitrogen and four ethyl arms, and the arms are not very movable. So it is basically a little pyramid that moves through the solution and can’t assume any very interesting conformations. And its similarity in size to a hydrated potassium was very suggestive. So the picture of the TEA lodging in a channel that was big enough to accept it as its inner end and (that) then narrowed down was immediately irresistible. And so after that, I guess I never looked back. It seemed that the channel had to be the way. But it was a long time before, I think, people were willing to accept this—that it was a channel rather than a carrier—because, of course, I guess it was the late ’60s early ’70s (that the carrier) Valinomycin was discovered. I’m a little vague on the precise date. But in any case it was potassium selective, and I think many people assumed that was going to be the answer to the potassium selectivity problem. We even managed with TEA to get a reasonably good estimate of the channel conductance, because one could measure the rate of entry of TEA if the concentration is sufficiency low. And then just by multiplying, scaling it from the concentration ratio (potassium/TEA), you could get an idea of how fast potassium was entering. And that turned out to be roughly one per micro-second. TEA, in fact, doesn’t seem to enter quite as readily as potassium, as we now know. But anyway, that gave an idea that the conductance of the conducting unit, which was still not known to be pore or channel, had to be pretty high, much more consistent with a pore than a carrier.
Baylor: Right, right. And I suppose the TEA then was in the back of your mind when you conceived the ball and chain mechanism for inactivation?
Armstrong: Well, yes, there was another step involved, and that was my favorite experiment, I think probably of all time, that I was involved with. And that was the TEA derivatives, which replaced one of the ethyl arms of TEA with a hydrophobic arm that was substantially longer. And nine carbons rather than two was my favorite…the triethylnonyl ammonium ion. I started lengthening the arms with the idea that I would perhaps learn something about the rate of entry because it should then depend on the orientation of the molecule. But the results were much more interesting than my expectation because it immediately became apparent that these were blockers of much higher potency (than TEA). They had such a high affinity for the channel that it was possible to see inactivation because they could be used at very low concentrations. And then (at low concentration) they entered the channel quite slowly and produced what certainly looked very much like sodium inactivation, but in a potassium channel. Well, there are lots of details in those experiments that I really liked, for example, that you could knock the ions out by raising the potassium concentration on the outside, having applied these things (TEA derivatives) to the inside. And well it all seemed to fit quite well with the general idea that the pore had a wide inner mouth and then narrowed so that the TEA derivative couldn’t work its way through, since it was not possible for it to lose its covalently attached arms. So that gave then a nice clue with regard to the selectivity mechanism of the channel, that the ion seemed likely to be dehydrating.
Baylor: Yes. So (regarding) the ideas about selectivity, I gather you interacted a fair amount with Bert Hille around that time.
Armstrong: Yes. I can’t remember precisely when I first met Bert, but it must have been in the late ’60s and after I had come back from London. And Bert was—well, I have very fond memories always of having interacted with Bert. He’s extremely stimulating and always a person of very great integrity and wonderfully imaginative. He and I were working to some degree on the same things. He tended to favor the sodium channel and TTX (tetrodotoxin), and there were his wonderful contributions on the actions of both of those substances (TTX and TEA) on the myelinated fibers. And then his great work on selectivity, which still provides the best model of selectivity of the sodium channel, and the local anesthetic work. And then there’s, of course, his great book, which is extremely influential.
Baylor: Yes. It’s really a wonderful source, isn’t it?
Armstrong: It is. It definitely has you might say paved the road for ion channels. Bert and I even worked together once upon a time in Seattle, which was a beautiful occasion. He took me out to the see the salmon there as they were running in the fall. And we applied some of the long chain TEA derivatives to the inside of a myelinated fiber and found out, in short, that they behaved pretty much the same with regard to the internal quaternary ammonium receptor, TEA receptor, like the squid axon. But the squid axon did not have an external TEA receptor at all, while the external TEA receptor in the case of the myelinated fiber, the frog myelinated fiber, was quite different in character (from the internal). It didn’t interact with the long chain compounds effectively at all. So it looked as though quite clearly there were two separate TEA sites, one at the inner end and one at the outer end, and they had different properties. And, of course, we now know that the external TEA sensitivity is assignable to a single tyrosine residue in each sub unit of the potassium channel, the work of Rod MacKinnon and colleagues.
Part 6: Ball and Chain Mechanism
Armstrong outlines his development of this mechanism for inactivation of channels, as well as his gating current experiments.
Baylor: I think the ball and chain mechanism for inactivation of channels was truly a remarkable idea. And it’s one of the few cases where somebody had such a radical idea that then turns out to be more or less exactly right. I’m still staggered that that worked so well. (Laughter.)
Armstrong: Well, I was also surprised, I might say. But obviously very pleased. The chain of thought was quite simple and that is having been presented with these experiments on TEA derivatives causing inactivation of the potassium channel and having found that pronase can remove inactivation from the sodium channel, when applied to the inside of the sodium channel, well it (sodium channel inactivation) certainly seemed easily explainable by saying that there is something analogous to TEA, some inactivating particle analogous to a long chain TEA molecule, that is tethered there at the inner end of the sodium channel. And we even got the stoichiometry of it right, by the way, from the pronase experiments because the time constant of the inactivation didn’t change. So it looked like one hit on each of the sodium channels, and that seems to be the case since (in) the sodium channel (inactivation) now resides by all accounts in the 3-4 domain linker.
Baylor: Right. So I guess an important part of the ball and chain mechanism was the idea that the channel had to open before the ball could go in and get stuck in the throat of the channel. And this was against what Hodgkin’s and Huxley’s picture had been and there must have been a fair amount of flack that you encountered as a result of that idea.
Armstrong: Well, pleasant flack. I mean it was flack from people who were basically extremely nice. But yes, it was…Well that was a big emotional step, too. I, of course, was convinced that it was right, but then I kept thinking well, you know, what is there to go on? So the first experiment to try and see if sodium inactivation had similarities was to see if one could see a lag in inactivation that was consistent with the idea that the channel had to open before it could inactivate. I managed to measure that on an axon down in Chile and had an abstract in the Biophysical Society in 1969 that, in fact, got the story more or less right (chuckles). It said that it looked as though two of the three Hodgkin and Huxley particles had to move in order for the channel to be able to inactivate. In fact, there is some inactivation apparently before the channel fully opens. But I didn’t really pursue that very strongly because I felt that it would be so easy for Hodgkin and Huxley to simply, let’s say, raise h (the inactivation variable) to the second power and thereby provide a lag in the inactivation. So I didn’t quite know what to do. The pronase experiment and then subsequently the gating current experiment turned out to be the way to proceed.
Baylor: Well, the gating current experiment was really wonderful because it showed directly that the gating charges do interact with the inactivation process and that charges become immobilized when the inactivation takes place.
Armstrong: Yes, that’s what we ultimately discovered. We started on the gating current experiments, I think along in the early ’70s. Of course, the existence of gating current was a prediction of the Hodgkin and Huxley scheme. If one thinks about it, there really is no alternative. If there is a voltage sensitive channel, then there has to be some charge movement associated with the structures that sense the change in the voltage.
Baylor: It’s interesting, though, that I remember in the early ’70s, before you found the gating current, Hodgkin saying that he thought that there ought to be a gating current. He couldn’t imagine how it would work otherwise, but he was so worried about whether it would really be there or not.
Armstrong: Yes. Well, there were several attempts to measure it. Knox Chandler and Hans Meves, for example, had made an attempt to measure gating currents. And then I think that if you look at one of their illustrations, they actually had it, but they thought they didn’t. But there is something that I think now one would call gating current. And Hans continued to work on it, and I think Richard Keynes was intending to work on it. And Eduardo Rojas was in England at the time, and so he communicated some of this fervor to Pancho and to me. And so we actually started working on it. We did our first experiment, in fact, with Eduardo, and I don’t think that one worked very well. Pancho and I got back to it sometime later and worked very hard, and that was an interesting, very lively time.
Baylor: Definitely. I remember the Cold Spring Harbor meeting in 1975 when you spoke about the gating currents and Eduardo Rojas spoke, and there was a certain amount of flack that went both directions. In fact, it was in some ways the highlight of the meeting that people could sit and see these two different groups with fairly different results.
Armstrong: Yes, well, it was stimulating, also somewhat embarrassing, since we were all supposedly professional, competent investigators, and we couldn’t get the elementary facts of the (gating current) behavior quite straightened out. But that, I think, must be the way these things go. I’m very proud to say that Pancho and I, I think, were right on all counts. And Richard Keynes at one time was extremely generous, I think, in basically admitting that. I wasn’t present at the Gordon Conference where he talked and said, I was told, that he’d come in sack cloth and ashes for Keynes and Rojas. But I thought that was really an extremely graceful gesture. Characteristic, I might say, of the general type of person who has always worked in the field. It’s a wonderful group of colleagues that I’ve had in working on ion channels. The pressure was really quite strong and many people were interested and wanted to know precisely, exactly how it worked. And that was a matter of detailed empirical description and not so easy to come by. So we worked for a long time and finally, I think, two of the major facts that we discovered were that the m system of Hodgkin and Huxley, the idea that there are three independent particles that move during the activation of a sodium channel did not seem to fit well with the turning off kinetics of the sodium channel. And the other was the finding that the gating current tails during the deactivation as the channels were turning off on repolarization, that those tails were not independent of inactivation as was predicted by the Hodgkin and Huxley model.
Baylor: Right. So the idea was that the ball was stuck in the pore and that this paralyzed the movement of the gating particles.
Armstrong: Yes, yes, right. So that fit perfectly well with the idea that some particle analogous to TEA had gotten into the channel and was preventing it from closing. And we subsequently found that, in fact, it immobilized about two-thirds of the charge. Also we were able to identify charge that, at least in theory, would make the channel nonconductive so that it did not reflux through the open state during recovery from inactivation.
Part 7: Zipper Idea
Armstrong comments on the origin and confirmation of this idea and concludes with encouragement for young scientists to enter the channel field.
Baylor: So how was it that you went from the charge movement, the thing that you measure with electrical techniques and stuff, and then came up with the idea of the zipper scheme for what the charge movement was representing structurally?
Armstrong: Oh, well, that was just, I guess, a lucky guess. After the gating current experiments and the quaternary ammonium experiments, I felt that inactivation was really fairly well solved from the conceptual point of view. I really thought that that had to be more or less right, that there was, in general terms, a conformational change that was associated with the opening of the channel and that that had to occur before something (the inactivating particle) could diffuse into the mouth of the channel and block it. And so that took care of an inactivation. It was clear from that experiment and from the TEA experiment that the gate had to be on the inside and it was, of course, abundantly clear since the time of Hodgkin and Huxley that the gate had to be voltage sensitive, and that that meant that there was charge movement, and now the charge movement had been identified. But to decide precisely how the charge moved was something of a problem. And so the zipper idea came from the notion that, well, one didn’t want the conformational change to be too large—that the idea of a particle moving all the way through the membrane seemed unattractive.
Baylor: Yes. So the minimal structural change that you could get away with and still have the gating current, sort of?
Armstrong: Yes, right. And that seemed to be best fit by the idea of having many charges moving simultaneously, each one of them through a small part of the electrical field. Let’s say if you had seven charges in a row, each of them could move a seventh of the way through the field for an equivalent electrical charge movement of one electronic charge.
Baylor: But this idea of the zipper then was, in a general way, confirmed very beautifully when the channels were cloned and when the S-4 region was identified. So this then formed a nice structural basis for the zipper.
Armstrong: Yes. I felt like someone upstairs was being good to me. (Laughter.)
Baylor: That, and then when Aldrich and his colleagues found the mutagenesis evidence for the ball and chain model, that’s again one of my very favorite confirmations of one of your ideas.
Armstrong: Well, that was a series of very lovely experiments by Rick and Bill Zagotta and Toshi Hoshi. Yeah, that was spectacular. So I had a double interest, both because it was such beautiful work and also because it seemed to fit this old idea of the ball and chain model. So that was lovely. Well, of course, the picture (in the channel field) has now changed completely, what with the patch clamp and cloning, and that provided wonderful new tools. The patch clamp has allowed us to look at all cells. No longer can you just look at the squid cell, squid axon, but any cell is now a reasonable target for electrical investigation. So that’s wonderful. And the cloning, of course, knowing the sequence of the channels, well, has lead to such wonderful things as the inactivation experiments of Rick (Aldrich) and his colleagues. And then the identification of the P region by MacKinnon and Gary Yellen, all starting in Chris Miller’s lab.
Baylor: And that’s all now moving forward at an ever-accelerating pace.
Armstrong: Yes, yes. So it’s dizzying to try to keep up with it. But the quality of the people working in the field is just staggering. I mean Rod and Gary Yellen and, well, Chris and Pancho and Rick Aldrich and Zagotta and Dick Horn and Isacoff and, oh, there are so many that I can’t name them all, but they’re all fantastic.
Baylor: It is really science at its very, very best. It’s a wonderful field.
Armstrong: I feel very pleased to have been in it.
Baylor: Definitely. Now would you recommend that a young person today go into this field, or in fact should a young person go into science? The type of science that you have done?
Armstrong: Yes! Oh, absolutely. What else is there to do? No, no, no, it’s great fun. It’s very exciting. Why would I tell somebody not to do it?
Baylor: It’s not too risky?
Armstrong: No, I don’t think it’s too risky. No, not at all. I mean do you know anybody who’s starving? Who is… no, you won’t make millions, but you’ll certainly live an interesting life.
Baylor: I agree. It’s a wonderful privilege.
Armstrong: After all, money isn’t everything.
Baylor: Right. Yet to find out a little something about nature is a wonderful privilege.
Armstrong: The Pleasure of Finding Things Out I think is one of Feynmann’s books, and it’s absolutely right. To know something that nobody else has known, it must be like being the first person to see, let’s say the first Westerner to see the Pacific Ocean. It’s just wonderful. And of course physical exploration is getting—there aren’t too many places that haven’t been seen now—but there are certainly many, many opportunities for exploring in science. And slowly, you know, increasing our general knowledge of the universe. It’s, well, it’s wonderful. So I would definitely say so, and also, you know, I would say to students that you don’t have to start immediately. Rod and I are examples of that (late starters).
Baylor: That’s right. Physicians.
Armstrong: Right. We started off as medical doctors. We didn’t have very rigorous training. I think in the case of both of us, we loved to look at physics books and try and figure things out, but we weren’t trained in it. So I think it’s never too late to start.
Baylor: So there’s hope for us all.
Armstrong: Ah. Well, yes, right. I think it is an extremely rewarding life, much more so than, well, I guess I better not use examples. (Laughter.) But I like it.
Baylor: So, Clay, it’s been a pleasure to talk to you about this stuff. It’s a fascinating insight into your career, and into the channels, and what a pleasure.
Armstrong: Well, Denis, for me being interviewed by you is an enormous pleasure. Someone that is such an accomplished scientist and someone with such an excellent understanding of all of these matters and such—well, your experiments, of course, are the admiration of the world. So thank you very much for taking the time to do this, Denis.
Baylor: Well, it’s my pleasure, and thank you, Clay. Look forward to talking to you again.
Key Publications by Clay Armstrong
Armstrong, C.M. (1971) Interaction of tetraethylammonium ion derivatives with the potassium channels of giant axons. J. Gen. Physiol. 58: 413–417.
Armstrong, C.M., Bezanilla, F., Horowicz, P. (1972) Twitches in the presence of ethylene glycol bis(-aminoethyl ether)-N, N’-tetraacetic acid. Biochim. Biophys. Acta 267: 605–608.
Armstrong, C.M., Bezanilla, F., Rojas, E. (1973) Destruction of sodium conductance in inactivation in squid axons perfused with pronase. J. Gen. Physiol. 62: 375–391.
Armstrong, C.M., Bezanilla, F. (1973) Currents related to the movement of the gating particles of the sodium channels. Nature 242: 459–461.
Armstrong, C.M., Bezanilla, F. (1977) Inactivation of the sodium channel. II. Gating current experiments. J. Gen. Physiol. 70: 567–590.
Armstrong, C.M. (1981) Sodium channels and gating currents. Physiol. Revs. 61: 644–683.
Bertil Hille interviewed by Eric Kandel
Eric Kandel, Senior Investigator, Howard Hughes Medical Institute, and Professor, Center for Neurobiology and Behavior, Columbia University, interviews Bertil Hille, who shares the 1999 Albert Lasker Basic Medical Research Award with Clay Armstrong and Roderick MacKinnon. Dr. Hille is a professor of physiology and biophysics at the University of Washington in Seattle.
Part 1: Growing Up At Yale
Dr. Hille recounts this early years at Yale as the son of faculty member in the mathematics department. At 16, a colleague of his father’s puts him to work in a science lab.
Kandel: I’ve outlined nine topics, and maybe I would just go through them with you and you can add and subtract, and then we can get into the actual discussion of these points. So I thought it would be good if you were to begin with a discussion of your life before the Rockefeller—a little bit about your upbringing, your family life, etc. Your father and mother, and your father’s influence on you; your undergraduate experience; and then of course Rockefeller, which was so important.
And that would lead into your first research project on excitable membranes, the sodium and potassium conductance pathway separation and would allow you to bring in the scene that you suggested we talk about, and that is online computing and development of your own instrumentation, which I gather you began at Rockefeller. And then on to the size and shape of the pore, that is, the different size organic and inorganic ion experiments. Then the selectivity filter, multi-ion occupancy, the modulated receptor hypothesis. Then maybe we would stop and speak a little bit about the interactions with Clay and Rod and then more recent work. And then perhaps end up with your thoughts about the influence on you and on the field of your earlier reviews and of the book. Does that sound reasonable?
Hille: Sounds terrific.
Kandel: Why don’t we begin.
Hille: I’m going to run out of voice before I get through all that.
Kandel: I doubt that. So tell me all about your youth.
Hille: My father was a mathematician at Yale and my mother, I would say, was an intellectual who was the wife of a faculty member. And in our household we always had scientists and mathematicians from Yale. Always people at the highest level. There were people like Lars Onsager, who invented irreversible thermodynamics. The Onsager family, his father and mother, were friends of my mother’s father and mother, and so that goes back generations. My father was a reader of his thesis. So there are many people who came through the house and were excellent scientists; the idea of science was just a given. My mother was also, although she wasn’t a scientist by training, she was also excellent in knowing causality and thinking about the laws of physics and making things follow from each other and being interested in all kinds of science.
Kandel: Are you an only child?
Hille: No, I have an older brother, who is now a linguist. He is a translator or terminology specialist at the UN. And he got his languages because my father was Swedish and my mother was Norwegian. They were both brought up in their home countries, and the first languages that we both heard were Norwegian and Swedish at home in the United States. Then we traveled to Europe many times, and we both went to school in France and in Germany and in Sweden, and in each case, we got another language and another culture. And that was a very European style of upbringing in a way. Instead of the other half of the world, it was just something we had lived in and knew how they thought in a way.
One of the new faculty members who came at the very same moment that my mother came, marrying my father, was Ed Boell who was a zoologist and embryologist at Yale. And he was a very good friend… they were very good friends of my family. And he said when Bertil gets to be 16, he can come into my laboratory. So when I was a kid at 16 in high school, I began something which maybe went on for six years, which was to work in Ed Boell’s laboratory at Yale. And that went on until I graduated with a bachelor’s degree in ’62. And so I sort of skipped adolescence and just went right to being a scientist in a laboratory, which for me was wonderful.
Kandel: So what sort of problems were you working on with Ed Boell?
Hille: He was interested in sort of development physiology. And so he wanted to see whether gills were important for the development of salamanders, whether they needed them. Whether they could survive at low oxygen tensions without gills. So I got these embryos and took off their enveloping membranes and put them in what is called a Warburg apparatus, which was a real ancient thing—a beautiful machine that measured how much oxygen they consumed. I also studied ion fluxes, which began me on the kinds of problems that I am still working on today. We had radioactive tracers and soaked the embryos, with and without gills and various things, in these different tracer ions. And I measured uptake and effluxes and then decided to make mathematical models describing saturation processes and all kinds of things, which I guess never came to anything, but it was good for a kid to learn how to do all that. It was very exciting and very formative for me.
Kandel: And how did you think of going to Rockefeller as a graduate? Excuse me one second before we get on that. So you presumably got your degree in biology at Yale?
Hille: Yes, so let’s go over Yale a little bit. At Yale, I took zoology as my bachelor’s degree. But I decided that I would do all the courses that were necessary for a degree called biophysics. And in the biophysics line their emphasis was things like thermodynamics, information theory, quantum mechanics, modern physics, relativity, those things. So I actually took rather nice courses in all of those subjects, which most zoologists wouldn’t do.
Hille goes to Rockefeller for graduate work at the suggestion of his mentor as an undergraduate at Yale. The “community of scholars” at Rockefeller produces many preeminent scientists.
Kandel: This was not the era in which Alex Mauro taught at Yale, was it?
Hille: Well, he was actually at the medical school at that time. And he was not teaching then. I think he was more or less taking care of electronics for Fulton and his crew. Setlow and Pollard were people who were at Yale at that time and had run the biophysics. And Harold Morowitz was an important person at that time. So through zoology, I got a love of, for example, evolution and learned the taxonomy of lots of kinds of organisms, something that always interested me and accounts for why at all times I seem to speculate about evolution of ion channels and what organisms used them for what.
So then I think we could go to Rockefeller. The reason I went to Rockefeller was that Ed Boell, who had taken me into his laboratory, wrote to Paul Weiss, who he knew, and Detlev Bronk, who was the president of Rockefeller, that apparently that I was an okay student and I should go there. So he told me about this letter, and I had never heard of Rockefeller, so I knew nothing about this. I was invited down to Rockefeller and Detlev Bronk spent the whole day, the president, with me while he was talking to David Rockefeller and running the National Academy of Sciences and running the university, all at the same time. And he spent the whole day with me, and then he said, okay you can come. And I said, well, I have to think about it. And when I came home Ed Boell said, what are you trying to do, play hard to get? (Laughter.) So basically I never applied anywhere else. I never applied anywhere. I went there because more or less my mentor said that is what I should do—which was terrific.
Kandel: Now this was the beginning of the graduate program at Rockefeller, if I remember correctly?
Hille: Well, it had been going for maybe five or six years. So there were a number of classes before me. In the earliest class were people like Gerry Edelman and Ed Reich and other people. So I was maybe the sixth class. This was actually a really unusual place, and it may not be quite the same anymore, but at the time Bronk had a very stylish way of having everything run. Everything was the best. The students had a very large budget, an infinite budget basically, and you could just go down and order on 8202, which was the budget number. Everybody will remember 8202. Whatever we wanted. Bronk called the Rockefeller a “community of scholars” and said that we were part of the community of scholars, so at once we were no longer students, we were just part of the community of scholars. And we always heard about many great people and the many Nobel prizes. And I took classes with George Palade and people like that. I did electron microscopy with him. And so we really were privileged.
Kandel: Who was in your class?
Hille: Well my class… there were 20 people in my class and six of us became members of the National Academy of Sciences. My closest friends were, for example, Harvey Lodish, who does cell biology at MIT; David Sabatini, who does cell biology at NYU; David Hirsh who is chairman of your biochemistry department.
Kandel: These are wonderful people.
Hille: These are examples of people who I, almost within the first week, became closely associated with, and our group was very highly intellectual and had a lot of different backgrounds. Harvey was a chemist, for example, at that time. He knew nothing about biology, so now he was supposed to study biology. So I gave him an enormous book of histology, which was by Ham and Leeson, I think. I gave it to him one night, and the next morning he came in and said, “That was a pretty good book. Do you have another?” (Laughter.) That was kind of the caliber of people who were there.
Kandel: Was Chuck in that year?
Hille: No. Chuck Stevens was maybe three years ahead of me. He was certainly there. Alan Finklestein was maybe three or four years ahead of me. Fred Dodge was perhaps five years ahead of me. But all those people were there still, and I knew them quite well and interacted with them.
Kandel: How did you focus in on your research project?
Hille: Well, interesting. I forgot to mention that at Yale, I was assigned a sort of senior honors advisor whose name was Tim Goldsmith, who a bit later became the chair of zoology at Yale. And so Tim (this was his first year at Yale too) didn’t talk to me much, but every once in a while would slip me a note and say, try reading this interesting paper. And then we didn’t talk about it afterwards, except he would say, “How was it?” And I would say, “okay,” and then he would give me another slip of paper. One of them was the Hodgkin and Huxley papers. So he just sent me off to the literature to read five papers by Hodgkin and Huxley. We never discussed it. It was kind of amusing. And I said it was okay.
And then he would give me a paper, let’s say in German, on the liquid junction potential by Henderson, and it would be from 1924. And I’d go off and find that somewhere and read that and say it was okay. Real eclectic, interesting stuff, which made me know the Hodgkin and Huxley papers before I came. In fact, Kacy Cole had come as a lecturer at Yale. So I heard him give several lectures which were really on the squid axon, including the Hodgkin and Huxley stuff. So by the time I came to Rockefeller, I already had some exposure to that. And I had, through my zoology training, sort of come to the conclusion that all of this was not really that interesting, that it wasn’t so interesting for biologists because it was a whole bunch of numerical and empirical curve fitting—events that biologists didn’t seem to be very interested in. And probably I should do something biological.
So I sort of rejected this area. But then I decided, well, membranes were really still interesting. So I tried to work on the sodium potassium ATPase, and I did that in Alfred Mirsky’s lab. And then in Palade’s lab, I studied holes made in red blood cells by complement, making lysis. And I did a variety of projects like that, but the people I was working with never got interested in them. So eventually I went back to being interested in the axons and their electrical excitability because Alex Mauro said, “Well, you know, if you don’t have anything else to do, why don’t you come to our lab, and you can study the effect of D2O on the propagation of impulses in lobster nerve trunks.” And that was great. We got a lot of lobsters and had a lot of bouillabaisse and a lot of parties, but it wasn’t really getting very mechanistic. Now this laboratory was the laboratory of Detlev Bronk, so it was the President’s laboratory. And it was peopled by Frank Brink, who was the dean of graduate studies; Clarence Connolly, who was the associate dean; and then Alex Mauro, Paul Hurlbut, and—I guess those were the people who were there.
So basically it was mostly an inactive laboratory. Of course it was all the deans and the president. The president never came, and the deans didn’t do any research, and Alex Mauro and Paul Hurlbut were doing some things. And Alex was, as you know, an eclectic person who had an interest in muscle satellite cells, in negative pressures, in osmosis, in thermodynamics and electrochemistry, in the history of Galvani and Volta and black widow spider venom toxins and later in Limulus ventral eye photo detection. So he had a lot of interests… it wasn’t any particular one thing that he focused on. And it was in that laboratory that Fred Dodge had done his voltage clamp work on nodes of Ranvier. And he had graduated just the year I came in and was now actually in Keffer Hartline’s laboratory studying the Limulus eye, which was on the same floor on the same hall. And Alan Finkelstein had just finished his work, and Chuck Stevens was graduating.
So Fred Dodge said, “You know, if you don’t like the D2O on the lobster axons, why don’t you use my old voltage clamp, which is sitting over here? We haven’t used it for several years, and it’s covered with dust, but we can make it go.” So he helped me fire up this machine with a whole rack full of vacuum tube power supplies and 300 volts positive and negative, and wound up all these things. There were a lot of things broken, and he just went in one after the other and fixed each part and set up things. And I gradually began to feel that if you were interested in electronics and you had to use it, you didn’t have to be some electrical engineer to fix it because here was Fred Dodge, who had just come from New Hampshire and wasn’t an engineer, and he was enjoying it, and it was very rational. So I got from this example the idea that one could work on electronics. In fact, I had taken my general exam at Rockefeller and one of the outcomes was that if I wanted to go into neurobiology, I better learn something about electronics.
So I learned a lot about that and from Fred’s lesson, I had actually learned a little bit of Fortran programming when I was at Yale and did some Fortran programs for the Hartline laboratory. In exchange they taught me how to do machine language programming, which we could do at the bit and byte level on their computer, which filled a whole room. They had a laboratory computer, one of the early ones, that took a whole room of space and had, gosh, I can’t remember, maybe 8,000 memory locations in it or something like that, and big magnetic tapes. So I learned from Fred Dodge how to voltage clamp nodes of Ranvier. And I photographed the pictures on graph cameras, as people did in those days, and projected the pictures under an enlarger on graph paper and tried to analyze the kinds of numbers that Hodgkin and Huxley were able to get and Fred Dodge and his mentor Bernard Frankenhaeuser in Sweden with whom he had done his original work. I got quickly bored, or discouraged, by how much projecting of film and measurement you had to make in the dark. And I decided that maybe you could automate this and use this computer which Hartline had in the other laboratory down the hall.
Part 3: The Saturday Project
Hille does all the experiments for his thesis at Rockefeller on Saturdays, the only day he was allowed to use the computer he needed for his work.
Kandel: So let’s stop here for a moment. Hartline was presumably not using it for online analysis. He was using it for data analysis after the experiment.
Hille: They actually were making online recordings. Their computer was new when I came, and you couldn’t buy an A to D converter and a D to A converter to make the recordings. They were designing them and building them. And they actually in the end put in sinusoidally modulated light into the Limulus eye and measured the voltage in the Limulus photoreceptor, which became sinusoidal. And these were recorded by the computer. And then they injected sinusoidal currents into the photoreceptor and measured the spike train that came out, which also had a sinusoidal component. They measured basically what was called the transfer function of each of the stages, and then they showed how as a linear system it operated. That’s the kind of thing they were doing. Actually what I wrote for them in Fortran was cross correlation analysis for them to cross correlate the sinusoidal inputs and outputs. But no one had ever done voltage clamp with a digital computer at that time.
Kandel: So this is the application based upon physical analyses.
Hille: The fact that the computer was there was perfect. It actually belonged to Hartline, and I was only allowed to use it on weekends. I did all the experiments in my thesis on Saturdays. And even when I came here to the University of Washington, I did experiments on Saturdays because the computer I was able to use here had the same deal. What I set up was a way to stimulate the node and record both the voltage and the currents and then to later subtract, or measure, from the records the leak current and subtract that, and measure the shape of the potassium current and fit that with the function, and then subtract that and leave the sodium current and fit it to rising and falling functions of the Hodgkin and Huxley type.
Kandel: I just want to step back for a second. If I remember the Dodge and Frankenhaeuser paper, they essentially confirmed for the node the Hodgkin and Huxley analysis of the squid axon, with the exception, I forget, there was no inactivation or there was less inactivation. I’ve forgotten the details, but it was pretty much showing that really a vertebrate nerve functioned in the same way as the squid axon. What specific question did you begin to address?
Hille: So right away… I just looked at my notebooks in preparation for thinking about this to see what I did. And the first experiment I did was to put on local anesthetics, Xylocaine (Lidocaine). And the next experiment was to put on tetrodotoxin, and the next experiment was to put on tetraethylammonium ions. And this was node number one and node number two and node number three.
Kandel: Isn’t that terrific! So maybe, for the person who’s not familiar with this, maybe you put this into some sort of a theoretical…
Hille: Why was I doing this? Well, I was in the laboratory of Alex Mauro, where he and Alan Finkelstein had been writing papers about electrodiffusion. I wasn’t clear exactly what they were saying, whether they were saying that they thought that all kinds of different ions could sort of go through the same regions of the membrane and they were showing how you would calculate the voltage and currents in that circumstance. And I thought they were maybe saying that in axons all the different ions were going through the same holes, not even holes, but just kind of the membrane itself. Like a piece of Kleenex, without any structure implied. And I personally thought that the currents carried by sodium ions and those carried by potassium ions must be going through different places.
Kandel: On the basis of what?
Hille: Well, so we sort of skipped over what we knew at the time. The Hodgkin and Huxley analysis had described the time course of an increase of sodium permeability and then its fall and then the time course of a rise of potassium permeability. And they declared that they couldn’t say anything about the structure of the membrane from this, but they could fit those time courses with mathematical functions and show that anything with those properties would be able to make an action potential because the rise and fall of sodium permeability and the rise of potassium permeability are exactly what you needed to make an action potential. So they had basically explained how you make the electrical signal of an axon—how you make it propagate—but had declared that no structural conclusion could be drawn from that. And now I think they felt, but they were reserved enough never to declare ever, I think they felt that most likely there are separate places for the sodium ions and potassium ions to go. A sodium-carrying mechanism and a potassium-carrying mechanism, which seemingly we began to call sodium channels and potassium channels. And that was sort of the issue with my perception of what Alex Mauro and Alan Finkelstein had done. It sounded to me like they were saying maybe these things go in the same place, and there certainly were many biophysicists who were saying that.
Kandel: Mullins… many people.
Part 4: Early Experiments
Hille details his work on axons that results in the first convincing demonstration that there are separate conduction pathways that can be molecularly distinguished from one another.
Hille: Mullins, in particular—a person who kept being the Devil’s advocate. He had nothing to lose, and he was a good protagonist for his point of view. He always pointed out the defects in the arguments. So it was sort of in that context that I felt that you could use pharmacological approaches or chemical approaches and decide that, if you could block or eliminate one of these two things without affecting the other, that they must be separate mechanisms. Hence my experiments with local anesthetics and tetrodotoxin and tetraethylammonium ions. Tetrodotoxin had already been described by Narahashi and Moore as blocking sodium conductance. The other ones were just sort of coming out of the blue. Clay Armstrong had a paper with Binstock in ’65, which was there first… on tetraethylammonium. My work was actually started before that. It came out after that. But tetraethylammonium ion and local anaesthetics and a long list of many other things had been in the literature as affecting conduction and shape of action potentials. And so what I did was to go back to—there was an enormous set of reviews, for example, by Abraham Shanes.
Kandel: I knew him well. Abe Shanes. Wonderful review.
Hille: Yeah, 140 pages long.
Kandel: Yeah, but terrific.
Hille: And he collected the effect of every drug that was known to work on axons. And so I just went through the list and I actually probably tried 100. And of those, you know, I happened to start with good ones right at the beginning. So those were my first experiments.
Kandel: But that must have been just a wonderful result. Did you and others appreciate how awesome the conclusions were that you could draw from that?
Hille: Well, immediately I declared it in my papers. The papers would always start out by saying—my papers—that we could block the sodium current with this and then the potassium current with that. And then when you got to the discussion, I would say, therefore, now we have separate places and we are going to call these sodium channels and potassium channels. That was the standard structure that I had. That to me was an important conclusion, and many people didn’t believe it, so it was still a big issue at that time.
Kandel: But that was probably the first convincing demonstration that there were separate conduction pathways that could be molecularly distinguished from one another.
Hille: Yeah, you could say that Narahashi and Moore in ’62, who had used the tetrodotoxin, you know they already had tetrodotoxin blocking sodium. And I showed it both ways. They actually didn’t believe it so much, so sometimes later they would publish a paper with an agent that blocked them both, and then they’d say, well, maybe they’re together. It wasn’t something that they firmly believed in. Clay Armstrong certainly believed in it already, and I believed in it already, before we started any experiments.
Kandel: Did you also conceive of the channels as being protein molecules? Was that clear at that time?
Hille: It wasn’t, no. An important set of classes that I had taken at Rockefeller was from Dan Koshland who was there. Actually he was at Brookhaven.
Kandel: Yes. He taught as an adjunct at the Rockefeller.
Hille: Yes, that’s right. So I took a course of theoretical chemistry from him, which I thought was terrific. And then I took enzymology from him, and that was terrific. So I had already had this training, which said that you could learn about the active site of an enzyme.
Kandel: And he was also very much interested in shape changes and allosteric mechanisms and conformational changes.
Hille: Exactly. Structure-activity and all those things. It was very clever. And how through chemical thinking, you could prove things. So I wanted to emulate that. This was a model for me even before I began experiments. These were things that I had heard about, and I said we’re going to do this to what’s in the membrane. But looking at my entire thesis, for example, I don’t think I ever used the word protein. And at the end of the thesis, there’s a drawing, which actually is going to be reproduced in Nature Medicine, drawing separate sodium and potassium and leak channels in the membrane and also an enzyme and also the sodium potassium ATPase—the only things we knew in the membrane. And they’re just sort of like little funnels, with nothing sticking out of the membrane, just the membrane kind of makes a funnel inside of it. There’s no substance. There’s no material, no chemical there.
Kandel: You know the discussion between Crick and Hodgkin that I think Hodgkin in his autobiography or Crick in his autobiography mentions, in which Hodgkin asked Crick, what kinds of molecules might mediate these currents? And he said, it has to be proteins! (Laughs.)
Hille: Now, I think if you asked me at that time, I would have said exactly the same thing. And certainly by the time I started my work in Seattle in 1968, I had proteins in my mind. But looking at my thesis I don’t see evidence that I really said proteins. I was telling you about Hodgkin, since I worked with him for a while and was associated with him. He said that you couldn’t write or talk about what you did when you were younger without actually going back and reading what you wrote down at the very same time, because you would always make yourself look better.
Kandel: Right. (Laughs.)
Hille: So I have to confess that I can’t say protein. It didn’t take many years though.
Kandel: Although the concept of channel had been around, you were the first one to actually use specifically sodium and potassium channels.
Hille: Actually in the literature and in my book, I mention about four papers in which the word appears once in somebody’s writing.
Part 5: Post Doc in England, On To Seattle
Hille works with Alan Hodgkin in England then finds a post at the University of Washington.
Kandel: As sodium and potassium channels? You trace the word channel all the way back to the 19th century, but I thought that—well, you tell me, go ahead.
Hille: In the Cambridge Group, Cambridge, England, which would be Hodgkin, Huxley, Keynes and those people. For example, in the Hodgkin and Keynes paper, which was 1955, about “it must be that the potassium channel has lots of ions in it in a row” they used the word channel once. They don’t say potassium channel, I don’t think. So it could be that Clay and I were… Clay certainly used potassium channel right away, without question. He started his terminology from the beginning of the paper. I would always be conservative at the beginning of the paper, and at the end of the paper I would be… “now we are going to call them… ” But he would just start right out. So he was clearly leading me there. Okay, so I think now we’re finished with my thesis basically, which was at Rockefeller, and maybe by now we’re… I had a year in England.
Kandel: That was right after Rockefeller?
Hille: Yes, I was postdoc with Hodgkin for one year.
Kandel: Now tell me what year that was.
Hille: That was 1967-68. And at that time Alan Hodgkin was doing experiments with Mordy Blaustein on the sodium-calcium exchanger. So I went to Plymouth, he sent me to Plymouth, and I was to study the calcium action potential that Tasaki had reported, that is an action potential carried by calcium ions. And I failed utterly to be able to do this. I was allowed as equipment only two cathode followers. He told me I couldn’t voltage clamp, and I wasn’t allowed to perfuse the axon. So I felt rather crippled.
Kandel: Why did he place those restrictions on you?
Hille: Well, he had the impression that experiments were very hard. And so, for example, when I was a graduate student after the internal perfusion of the squid axon was published by Baker, Hodgkin and Shaw. I wrote a letter to Hodgkin, after I had learned how to voltage clamp, saying this seemed like a wonderful technique, and the idea of combining perfusion of axons with voltage clamp would be wonderful. And actually I have a letter right here in front of me. He wrote back and said, “The technique is formidable, and I rather doubt whether anyone in Plymouth will get it going again in the immediate future.” Actually he had told me that Knox Chandler and Hans Meves were doing that technique at this very moment, as he was writing this letter, in Plymouth. But then he was telling me that it was too hard to ever do again. So somehow he had the impression that experiments of that kind were so difficult that one couldn’t do them. Interesting. Kenneth Cole told me when I wrote him that I was going to have a year with Hodgkin, he said, “I should warn you, Hodgkin doesn’t like voltage clamps,” and he was right.
Kandel: That’s amazing. It’s absolutely amazing.
Hille: And it could be some kind of caution, that he thought that the experiments required a genius like Huxley to be there to be sure that they were done right. And that if you did something, you would probably do it wrong, and therefore, it wasn’t worth doing. I’m guessing that that was sort of the context of this. Larry Cohen was actually in Plymouth at the time beginning optical experiments, and he had found a birefringence change, and I had actually made a voltage clamp surreptitiously for the squid axon, even though I was told not to. And Richard Keynes heard about that. So he said, you know, why don’t you stick your voltage clamp into Larry Cohen’s axons and we can do birefringence with voltage clamps. And I switched over to doing that. And I continued actually that year working mostly with Larry Cohen in Richard Keynes’s place. So I spent very little time with Alan Hodgkin. Actually when I finished at Plymouth, Alan Hodgkin told me that there was no place for me to work in Cambridge, so I would have to do a theoretical problem. That was one of the bases of my continuing in Babraham, because I had no theoretical problem in mind what so ever.
Kandel: So you came back to the United States in ’68?
Hille: Actually, while I was a graduate student, before I had defended my thesis even, I had made a tour of the United States, especially the West Coast, looking for jobs, and I went to maybe seven institutions on the West Coast. And I liked Seattle, University of Washington, the best. Several places gave me offers.
Kandel: You told me once I think, many years ago, that one of the reasons you liked Seattle is because Woodbury had computers. Is that right?
Hille: It’s exactly right. Because I had more or less decided that it was necessary for me to have an online computer to record my experiments or else I couldn’t do them. And it wasn’t actually Walter Woodbury who had them, it was another person who was making big computers in the department, but they were here and they knew all about what that was.
Kandel: And they committed themselves to you that you would have access to it.
Hille: Right. On weekends. (Laughter.) And then I could also use them for an hour or two here and there to analyze. But to do experiments you have to have a lot of time. So I did those on the weekends, too. So really, you’re right, it was because they had computers. When I did this trip—interesting what those days were—it wasn’t because any place had an advertised job. It was because Alex Mauro had written to these places and said, “My student is coming by, and he’d like to give a talk. And he’s looking for a job.” Several places wrote back and said they didn’t have jobs, but I could come. And I wrote Chuck Stevens, who was here, because I had known him as a student—and the same thing, he said no job. So I went to all these places and almost every one of them offered me a job. So in those days, jobs just materialized if they had somebody in mind, and otherwise there was nothing. You didn’t have to advertise or compete, it was just a matter of did they want some such person. Nobody paid my airfare or trip expenses except for Simon Fraser University in British Columbia, which paid the airfare from Vancouver, British Columbia, back to New York. Nobody promised me any startup money. So I accepted the deal in Seattle without their having paid any of the expenses—I actually stayed in Chuck Steven’s house—without any startup money; no statement about how much teaching or space I would get, but I would get a room somewhere. And you just shake hands on that, and that was it.
Kandel: You should negotiate all over again. (Laughter.)
Hille: Yeah, okay. So when I came here, the first thing I decided to do was to measure the conductance of single channels, or to work on that problem, which was a problem which wasn’t really solved until patch clamping came out, although noise did something good for it. And I spent a frustrating year working on that question, and finally just threw out everything.
Kandel: So I’m sorry, this is something I was not aware of…
Hille: Because it’s not published.
Kandel: That I’m aware of (laughs). But how did people think about single channels in ’68? Did they think about it?
Part 6: Related Studies
Hille comments on papers by other scientists related to his work on composite currents.
Hille: Well, in my thesis in ’67 and in a paper I published in ’68 as an appendix, I calculated the conductance a single channel could have on the basis of diffusion to the channel, on the basis of resistance that a hole would have if it was small enough to hold an ion, and things of that kind.
Kandel: So, were you the first one to begin thinking about the fact that these were composite currents and that one could think of them as being made up of lots of channels?
Hille: Well I don’t think so, but I actually believe I’m the first one to try to write down numbers in the literature that said, this is what the conductance of a channel might be, and this is, therefore, how many channels you might have in a node of Ranvier because of that.
Kandel: How did this relate to the period in which people were doing artificial membranes?
Hille: Okay, well yes, that’s a good point you’re making. Hladkey and Hayden actually were working on gramicidin. I met Stephen Hladkey in Cambridge when I was a postdoc, and they were just starting those experiments. So probably by the time I came here they had published a single channel gramicidin paper in bilayers—in ’68 or something of that time. Also Katz and Miledi had begun to…
Kandel: But they didn’t publish until the 1970 or 1971. The noise analysis. And they began to think of that for the ACh receptor, sure.
Hille: But my writing about the conductance was probably before that stuff. And I think I was a factor of three off. What I calculated mostly was the limiting possibilities. You can’t go higher than 300 picosemens, I said, and that’s about the highest channel that we know of even today. And it turned out that sodium channels are somewhat smaller. So anyway, I tried to do this, and I was completely fooled by… It turned out if you looked up on a random number table, you could get data that looked like the data I had. And if you binned them into histograms you would see periodicities that would make you think that you had sort of modes of their stepwise increasing of an electrical property or any property that you measured. And I was completely fooled by what you could find in a random number generator and making a histogram from it—that you get periods. I was looking for the effects of 1 channel, 2 channel, 3 channel, 4 channels kind of thing. And so when I finally had the sense to use a random number generator to compare my experiments with, I realized it was junk. So then right away, the next day, I looked in my notebook and it says, “The conclusions from this set of experiments are”—and then in red—”zilch.” The next day I mixed five different ions in solutions and began to study selectivity, and that worked.
Kandel: So this was clearly in the back of your mind.
Hille: And it was also in my grant proposal. In fact, I had even done some as a graduate student. As a graduate student perhaps the best paper that came out when I started was the paper by Chandler and Meves, where they had done this work that Hodgkin said was going on in Plymouth—perfusion—and they showed that the sodium channel of the squid axon can accept lithium, sodium, potassium, rubidium and cesium and gave permeabilities for each of those. So soon after that, I did the same experiments, a little bit, myself at Rockefeller. For example, lithium is published in at least one paper of mine. So I knew that you could do it, and I did this in earnest at the University of Washington.
Kandel: If I remember correctly the Meves-Chandler paper did not use organics. They used a very limited range.
Hille: They just used those five. Now in the literature there had already been papers by Koketsu and Tasaki.
Kandel: They tried everything—in some haphazard way.
Hille: And all of these authors had shown that if you take away the sodium…
Kandel: You could substitute.
Hille: You could give it guanidinium or something else.
Kandel: They did it in order to make Hodgkin look foolish.
Hille: That’s right. So their purpose was to refute the sodium theory, and my purpose was to take their interesting results and interpret it as those ions going through sodium channels, which I did. Actually somebody, I think Ehrenstein and Gilbert at the NIH, had already published a paper, I think at that time, showing that ammonium would substitute for potassium in the squid ion axon, perhaps in the potassium channel and maybe also in the sodium channel, and from those kinds of experiments then they made some speculation about how selectivity works, because ammonium has a four-fold symmetry or a tetrahedral symmetry or whatever. And I decided that you couldn’t really do it on the basis of just one other ion, you had to take every possible ion, every ion that existed and see what the results were before you could sit down and make what your theory is.
And so I started with ammonium and then amino-ammonium (which is hydrazine), hydroxyl ammonium, methyl ammonium, fomamidine, amidine, guanadine, methyl guanidine, hydroxyguanidine, aminoguanidine. I just looked up, mostly it was Eastman Organics and Aldrich catalogues. I kind of wrote down, okay, you put another nitrogen next to this nitrogen, what do you get? And I would look up what’s the name of that and look it up in the catalogue. Or I would just thumb through the pages and look for pictures and say, “Oh, that’s an interesting compound.” So I believe that I tested every compound that exists, which is smaller than the sizes that didn’t go through the hole. And so finally I had what I felt was the comprehensive list, and then I had to explain, what does it mean? And that turned out to be pretty hard. The greatest difficulty was that methylammonium did not go through the sodium channel, and yet something like aminoguanidine, which sounds a lot bigger and is sort of a lot bigger, does. So how can you make a hole that does accept the big one and doesn’t accept the little one?
Part 7: Breakthrough Publication
Hille works for a year to formulate his conclusion that the hole was only one of the defining features in selectivity.
Kandel: So that’s how you came to the conclusion that the hole was only one of the defining features in selectivity.
Hille: Well, actually, one struggle that was going on at that time was people had, up until that time, wanted the hydrated ions to be what goes through the hole, and the hydrated ions were a big fuzzy ball surrounded by water. And I was looking at my data, and I thought, you know you couldn’t possibly know what was inside the fuzzy ball at the level of what’s the difference between hydrazine and methyl ammonium. They’re virtually identical, so similar. How could you know? So I thought you just had to take the water off because otherwise you couldn’t see what was in there.
And so I took the water off and made the hole small. And then I realized that if you allowed amino groups, which have hydrogens on them that can hydrogen bond, if you make a hydrogen bond and you buy the CPK models of chemistry and you build them, you see the hydrogen bond. Actually the amino group gets much closer to the group that it hydrogen bonds to than it would if the hydrogen was built with the full round radius of one Angstrom that a hydrogen has. Hydrogen bond means you shorten the distance. So I thought, well, if you make the channel out of material that accepts hydrogen bonds, then those molecules that can make hydrogen bonds will look small, because they can get much closer, whereas a methyl group, which can’t make a hydrogen bond, doesn’t look small anymore. And that was the trick. So I made a hole that was so small that a methyl group couldn’t go through, but anything that could form a hydrogen bond, like an ammonium, looked small so it could pass through, even though if you don’t have a hydrogen bond it looks just the same size as a methyl group. So that was really…
Kandel: That’s remarkable insight.
Hille: Yeah, and it took me a year. I mean, I sat with these data for a whole year while I just sat and wrestled with the problem of, do I have to take all the water off? So here is a case where I just had all this stuff in front of me, the data, and you couldn’t publish because I didn’t know what to make of it. It took a long time just to think about it. And then the first paper that came out promised four more papers—I just read it today—which were papers that came out over the next four years on a variety of subjects that we’ll be getting to shortly. So those two papers, actually, defined the shape of the pore on the basis of criteria that no one had ever used before. And you had no way of knowing whether it was the right answer because you didn’t have anything whose structure was known for which those arguments had been used. So it was a hypothesis that just has sat there. In the following year, I did it for potassium channels as Clay Armstrong and Bezanilla also did. And we both came to the conclusion that it’s about a 3 Angstrom hole on the basis of the same kind of arguments. And that’s what Rod MacKinnon has shown us in his crystal structure of 1998. And so for the first time, we had a calibration that maybe that method does work. I hope it works for sodium channels, too. We don’t know that yet.
Kandel: (Laughter.) We don’t, but very likely it will.
Hille: So that was defining the size and shape of the hole, for which we don’t yet know if the answer is right. We only have this logic. At the same time, I found that a certain ion would go twice as well, or half as well or whatever, as sodium ions by the criterion of measuring the reversal potential, which is the voltage at which the current goes to zero. Let’s say you had sodium ions on both sides, if you replace the sodium ions with a test ion, you now have to go to a different voltage to keep the net current from going outwards or inwards or it doesn’t stay the same. So there’s a criterion for measuring permeability, which is the one I used first, which was on the basis of this voltage as predicted by the Goldman Hodgkin voltage equation. But while I was doing it, it was obvious that the ions that I said were half as permeable as sodium ions often gave only 1/5 of the current or 1/10 of the current. So the size of the current gave a different answer for what the relative permeability of the ion was in comparison to the reversal potential.
And this is getting very technical now, but it was bothersome because in the theories that Hodgkin and his followers had written down, those things should be exactly the same. Those are theories based on what they had called the independence principal. And so I had to wrestle with what was going on. In the first papers, I wrote that the ion acts like an anesthetic. It seems to reduce the number of channels working, while also being a permeable ion. So then I began to think about the ion entering the channel and just sitting there a long time. So if the test ion goes into a channel and sits a long time, if it, let’s say, in competition with sodium ions, goes into the channel just as easily as a sodium ion, but it sits there a long time and doesn’t come out the other side until later, then that ion will certainly carry small current, because it sits in the channel a long time and the channel is tied up. Like having an accident in the tunnel.
On the other hand, from the voltage criterion you could show that this kind of situation where the test ion goes into the channel just as easily as sodium but it stays a long time, it on the voltage criterion would come out to be equally permeable. So then I began to understand how you could get a difference. And to describe that, I decided to use Eyring rate theory, which is the idea that ions jump over barriers and sit in wells and jump over another barrier and sit in a well, as they are proceeding through the channel, not quite like free diffusion. Now Eyring rate theory is something that I had already learned at Yale in biophysics. So in my biophysics major it was one of the things that we did. And at that time I had even read a paper by Walter Woodbury in which he had applied it to axon permeabilities in 1949. So Walter Woodbury has a paper back then.
Kandel: It’s funny, because I always had the feeling that you were one of the earliest people to apply that.
Hille: Well, so there is actually a literature in which Henry Eyring himself said the Eyring rate theory will do for anything, so it will do for diffusion, for example. And Walter Woodbury was probably a post doc in Utah with Henry Eyrring and became interested in nerves, and he wrote an article about that. Now in his article—it’s probably Eyring, Lumry and Woodbury or something like that—in his article, they didn’t have the idea that the ion was staying a long time in the pore. They just used the barrier height as a way to get higher permeability or lower permeability. So it didn’t do the thing that I was trying to describe.
Kandel: Energy barriers of various kinds within the channel.
Part 8: Further Research
Hille writes a paper on the various things that would happen if you had a long pore, as described by Eyring rate theory, in which you allowed multiple ions to be present in the different sites, but they couldn’t go by each other.
Hille: It had energy barriers, but it didn’t have the wells which retained the ions. So the structure was there for thinking about it, but no one had actually applied it the way we did. I wrote a paper on describing sodium channels that way, and for that paper, I assumed that only one ion could be in the channel at a time. So if there’s an ion there, no other ion can go through. And that described what we called deviations from independence—this paradox of two kinds of permeability in sodium channels. And then later, Wolfgang Schwartz, who joined me as a postdoc, and I tackled the harder problem, which is what happens if you have several ions in the pore at once. It’s just harder from the mathematical point of view. Which was something that Hodgkin and Keynes had suggested for the potassium channel—they had some big number of ions.
And so we wrote a paper on, or did a lot of thinking on, that—it took a long time to think about that—and wrote a paper on the various things that would happen if you had a long pore, as described by Eyring rate theory, in which you allowed multiple ions to be present in the different sites, but they couldn’t go by each other. They had to wait for the next one to move out of the site before you could jump into that site. And we described what Hodgkin and Keynes had first described, which was their so-called flux-ratio experiment with isotopes going in and out. That is what led them to this conclusion in the first place. But we went on to show that you could get other phenomena, like if one of the ions was a blocking ion that could go down a certain number of sites, but just couldn’t get over the last barrier, let’s say, it would block the channel if it was pushed down into there, you could get quite unexpected high voltage dependence of that block.
And this we suggested could be used to explain how channels rectify—inward rectifier channels or potassium channels that don’t let potassium go out of the cell, but let potassium ions come into the cell. And if you decided that there was a blocking ion in the cytoplasm that would go into the channel and plug it up and the channel had multiple ions in it at once, then you could get the peculiar phenomena that are known for inward rectifiers. You could make the current size depend upon the concentrations of potassium on the two sides. You could make the block very voltage dependent, and so forth. So that paper used Eyring rate theory again. We sent it to the Journal of General Physiology, and they couldn’t decide about it. It took five reviewers.
Kandel: They didn’t understand it. (Chuckles.)
Hille: Well, no, they could understand it. There was, of course, the debate about whether a theoretical paper deserved to be in the Journal of General Physiology. But there was also the debate about whether the potassium channels really were pores, because even in 1978, which was this time, there are models of inward rectifiers, for example—which are potassium channels—that worked well when they were carriers. And so I think the overall resistance was that, you know, here we were giving sort of unfettered salesmanship to the idea that all potassium channels are pores, acting as if it was something we could just sort of start with when we didn’t even know it. So I think that was one of the last times that I remember encountering complete resistance to the pore theory.
Okay, so I think that covers our sort of physical chemical exercises. There are others into what ions see when they go into the pore and how they feel when they go through it. Now I should say, all of this actually dates back in a way to Clay Armstrong’s first research, which was tetraethylammonium ions block potassium channels by entering from the inside. They go into the channel, they plug it up, and potassium ions can push them out. And these ideas, which he started with in 1965 when I was just beginning my own experiments, are ones that came into my own work from him, all the time… repeatedly.
Kandel: Maybe this would be a good time for you to discuss a little bit about the actual, you know, interactions between the two of you and the influence that might not have been dependent on your talking to each other—or were the two synonymous? Did you talk to each other a great deal or did you primarily influence each other by reading each other’s papers?
Hille: I guess both actually. I looked back at that question also to try to figure out what we had done, and I found a letter from Kenneth Cole to me in 1966. I had sent …
Kandel: Now Clay was Kenneth’s student, was Cole’s student, wasn’t he?
Hille: Clay was at the NIH. Clay was an M.D. And I think at the NIH basically you could do your residency, you know, as a scientist instead of…
Kandel: Yes, I was there also. So you could take a postdoctoral fellowship with somebody there.
Hille: You didn’t have to go be in the war or whatever it was.
Kandel: It was not a substitute residency, it was a substitute for the draft.
Hille: There we go! (Laughter.) You know better than I. So Clay was in Kacy Cole’s lab at the NIH doing this. Also FitzHugh and I think Knox Chandler, Bob Taylor and a few others were there at that time. So I sent my draft of a paper, my first paper on tetraethylammonium ions, which I wrote in ’66 and was published in ’67, I sent it to Kacy Cole. I actually met Kacy Cole in ’65, and it turned out we corresponded almost every month from then on, surprisingly. He was a very active corresponder.
Kandel: For how long?
Hille: Oh, until maybe ’71 or something like that. Lots of back and forth. I have to tell you how I met him. I met him because in the summer of ’65, I worked, in the summer I worked in Harry Grundfest’s lab in Woods Hole, and I did that because I liked Woods Hole. And I asked Alex Mauro, how can I go to Woods Hole? And he said, well, I know Harry, and we can do that. So I interacted with Kacy Cole in Woods Hole, and he was interested in what my conclusions were of that summer’s work, and we talked about it. So I sent him my work on tetraethylammonium ions in ’66, and he wrote back and said, “It’s interesting in this way and here are problems with your grammar and so forth, and Clay Armstrong is doing some work that’s closely related. You should talk about it, and I shouldn’t be the one who tells you about it. Instead why don’t you send your paper in to Clay and maybe he can send you his.”
So I saw a letter there that said, “Dear Dr. Armstrong, I am sending you my manuscript at the suggestion of Dr. Cole.” So that was in 1966. I think that was really the first time—I had read his paper in ’65—but the first time I interacted. And then by ’68, ’69, ’70, Clay and I were corresponding quite regularly, and I reviewed every paper he published for ten years for the Journal of General Physiology, and he probably reviewed mine starting in ’69. So we had lots of interaction. Then in 1970, he wrote me a letter in the summer and said, you know there was a paper in Pfloger’s Archiv from Vogel and Kopenhoffer that said if you cut the ends of a myelinated nerve fiber in tetraethylammonium ion, it will go inside and block from the inside, and it looks different from blocking from the outside. And he proposed that we do some experiments together.
Kandel: You collaborated with him.
Hille: Yes.
Part 9: Collaboration and New Line of Studies
Hille talks about his collaboration with Clay Armstrong and his later work on local anesthetics.
Hille: And so by November of that year, in 1970, he was in my lab and we spent two weeks here doing experiments together. We published together, and it came out in 1972. We explored the idea that tetraethylammonium ion was blocking from the inside, waiting for the gate of the potassium channel to open, and this would show that the gate was on the inside side and so forth, which were the same themes that he was developing in the squid. And then I found letters which said, “Clay, I thought your manuscript was wonderful,” and so forth, where we were trading manuscripts with each other and criticizing them. I think when we saw each other in 1970, we both took care to tell each other that we both thought the other person wasn’t doing interesting experiments anymore and ought to change to some other subject. (Laughter.)
I think I told him basically that TEA is pretty well finished, why don’t you do something else. And I had already published my first paper on selectivity, which Clay was careful to tell me was a pretty weak paper. It wasn’t the good paper, actually, it was a sort of preliminary paper in PNAS that Kenneth Cole had sponsored for me. So anyway, we had an influence right from the beginning. And certainly reading Clay’s paper of 1965 after I had done two months of experiments with TEA, you know that was very influential as an interpretation, it turned out that he was studying TEA that acts from the inside, and I was studying TEA that acted from the outside. Actually one of the reasons he came in 1970 was that he didn’t believe my idea that it was on the outside. And what we ended up finding was there is a receptor on the outside, and there is a receptor on the inside. And so then, everybody was happy with that. (Laughter.)
Another substance that blocks from the inside is local anesthetics. And even in my thesis—as I said the very first experiment I did on the computer with the node of Ranvier was with Lidocaine. It turned out that Alex Mauro, who was my thesis advisor, knew George Camugis, who was in Astra Pharmaceuticals in Massachusetts, and so therefore, we had a supply of Astra compounds of which Lidocaine was one. And we even went up there and talked to them, and they made some more compounds. So for ten years I had a good interaction with the Astra people on getting various derivatives, analogs of local anesthetics, which might test better some concept that I had. So local anesthetics blocked sodium channels. I got a quaternary anesthetic, which would be a charged one that doesn’t go through membranes, and Narahashi had shown that putting those inside the cell blocked sodium channels.
So I gave that to my first postdoc, who was Gary Strichartz, and Gary put this on the inside of node of Ranvier, cutting the ends the way Kopenhoffer and Vogel showed you could let it diffuse to the inside of the node. And then he started telling me that if you stimulated the axon repetitively, the amount of block started to increase. The sodium current would get smaller and smaller and smaller each time you stimulated it. I said, do it again. So he would do it again, and he said same answer. And I said, look, I’ll do the experiment with you and show you how to do it. And it still did that! So that was the origin of use-dependent block, where apparently just like the potassium channel, there’s a gate on the inside, a quaternary ion, in this case the local anesthetic analog, waits until the gate opens and then it can go into the channel.
So that began an interesting many years of experiments with local anesthetics which in the end after working with quaternary ones, I decided I was going to try to figure out again what form of the anesthetic was active in the free amine. Was it the charged form or was it the neutral? So I did a lot of experiments with pH changes, which were to change the ionization of that group. And it’s those experiments which led me finally to what I called the modulated receptor hypothesis. And that idea—which once again is really just an extension of Clay Armstrong’s first idea with potassium channels from his very first papers—the idea was that the reaction of the local anesthetic molecule depends upon the gating state of the channel. If the gate is open, it can go in or out. If the gate is closed, it can’t move. And I added, if the channel is inactivated, which is yet another gating state, the anesthetic binds more strongly. Then I decided that ionizable anesthetics, which have a neutral form, can actually come and go from the channel even when it’s closed… that they can get into the channel through a route which isn’t through the gate, unlike the quaternary ones which are completely trapped.
So I developed the idea that anesthetics—bound to the channel more strongly when it was inactivated—so I developed a model where there were three gating states, the resting channel, the open channel, and the inactivated the channel, and each one had a different affinity for anesthetic. If it was a quaternary anesthetic, it was limited by the gate closing to not moving at that time, and it could only move when the gate’s open. And if it wasn’t quaternary, it would come and go through the membrane into the channel pore, somehow. And I said, the neutral and the charged forms are both active and binding and blocking in the same place. That was the modulated receptor hypothesis. It would lead the channel to become more and more blocked if you stimulated rapidly. That’s because the channel would become inactivated and bind the anesthetic more and more firmly.
Kandel: I just wanted to make sure that you would discuss two implications of that as you are talking about this. One is the fact that, if my memory serves me, this is the first introduction of the inactivated state as being a discrete state that had significance beyond just inactivating the channel—that it affected how ligands bind to the channel. And the second thing is, it indicated that the gating conformational change is quite widespread and affects the whole extent of the molecule, if you will.
Hille: Yeah, okay, and then there’s a third thing that we’ll get to, which is how this relates to antiarrhythmias, antiarrhythmic action.
Part 10: Modulated Receptor Hypothesis
Hille elaborates on the inactivated state, the gating conformational change and antiarrhyhythmic action.
Kandel: Three points of the modulated receptor hypothesis.
Hille: So one point you made was, it brought in the inactivated state as a real state, and this state somehow has a global influence that isn’t just whether the ions can go through, but it does something else. And those same ideas were coming out also from work with scorpion toxins. So it turns out that a peptide, the scorpion toxin, can bind from the outside. This is work that was done in my laboratory actually by Mike Cahalan in ’75. It combines from the outside and affects inactivation, and the amount of binding was affected by what the membrane potential was and whether you depolarized the cell. So there in parallel, all these things were happening sort of at the same time.
Here was a peptide, a big molecule that could bind to the channel and seemed to know on the outside what its state was, and affected this state call inactivation. So there’s kind of another global thing. So within the lab this was now becoming common and easy to accept. But it’s true, the kind of experiments which Clay initiated, that gates affect the binding drugs, added a new dimension to what gates must be, because it wasn’t just whether an ion goes through, it was a whole bunch of other properties that you could now see. And today people tie fluorescent molecules to different parts of the channel and watch the fluorescence change when the gates change. They can see the same thing even better—more beautifully.
Okay, so this work on anesthetics: Throughout, the reason I did it, was because I thought it would tell me the shape and size of the pore. Something about the mechanics, and where the machines are, and what the gizmos are inside the pore. And I was completely oblivious to the fact that cardiologists were using these same compounds to control arrhythmias of the heart and that in arrhythmias, when you had too early or too frequent action potentials, the drugs would work more strongly, which is really the same as the use dependent block by local anesthetics. We published our work without any reference to that concept, and at the very same time Hondeghem and Katzung published a paper which was sort of a theoretical paper about arrhythmias and the action of antiarrhythmics, which are these same kinds of compounds, on sodium channels.
And it turned out, basically, we published the same ideas contemporaneously. And this, the fact that it related to cardiac things, meant that suddenly I was being invited to meetings where either anesthesiologists or cardiologists were involved to describe this work, which was very relevant to them. But I have to confess, we didn’t do it because of them. It was a kind of serendipity of basic science, or our blindness maybe even, coming up with a result which we should have known was interesting to them. But we weren’t doing it because of them, and we didn’t know about them, interestingly. Because it relates to those things, the two papers I published then are actually some of the most highly cited papers I have. I have hundreds of citations.
When we were mentioning the pore size, we didn’t go on to the pore size of the nicotinic acetylcholine receptor, which was later work that comes about now. This is around 1979. And I got the idea that since people were beginning to do biochemistry, and eventually cloning, on channels that it was a good idea for the physiologist to tell the biochemist what the properties of the channels were so that they could look for that. And it would be embarrassing, I thought, if the biochemist told the physiologist what the channels were.
So I said, you know, people like Arthur Karlin and Jean-Pierre Changeux and those people, they’re going to get the nicotinic acetylcholine receptor. We better find out about its pore, because it hasn’t been described well. So Terry Dwyer and David Adams did a whole big series of experiments doing the same thing as I did with sodium channels and potassium channels, looking up every ion—I did the looking up of the ions, they did the tests—every ion that would be of a certain size, see if it goes through. And in this case, we bought and tested 50 ions before we found one that wasn’t going through. And then we tested another 25 or 30 more that didn’t go through, but it’s a big job. They did a lot of work.
Kandel: It was a big hole.
Hille: It was a big hole. It surprised us.
Kandel: Yes. It was astonishing.
Hille: We got another methyl group and another methyl group and another methyl group, and it just kept opening its mouth and saying, sure, I’ll take that. So I had bought already the CPK models, the chemistry models for making model molecules, and I built all the ions as, all the bigger ones. And I took a piece of Styrofoam and addressed it with these molecules. And I cut with a scalpel the hole, and then I would stick another molecule into it, and if it wouldn’t go through the hole, I’d shave away some piece until it would. Then I’d do this with every one of the ions, and finally I had a hole that everybody would go through. And it was about 6 by 6 Angstroms, which is a big hole.
Kandel: Gigantic yes.
Hille: So there’s a case where actually if we are talking about sodium and potassium, they can go through with lots of their waters. Those are the ions that would normally go through. Okay so then, again because of the biochemists coming into this work, I felt it was time to write up for the world, for biochemists and others, what we knew. So this was writing my book.
Kandel: I see, so you had in mind in fact that the field was beginning to be invaded, as it were, by people from other disciplines who needed guidelines to the structural characterization of ion channels.
Hille: Yeah that’s sort of, I mean, I put it in a slightly negative light. There were two things. There were new people coming into the field. Patch clamp was just being invented, so that also attracted a lot of people, and I felt that classical work had determined a great number of things. And I was already finding that the people who were doing biochemistry on the nicotinic receptor—not Arthur Karlin, but others—were discovering, let’s say, desensitization of the receptor and giving it a new name and not knowing that there was such a thing that had a name and that had a model and that Katz and Theslef had tested and had made a nice description of it and so forth. So these were the kinds of motivations that… There were new people coming in and they couldn’t read this literature, actually, which was pretty complicated. It’s difficult to read the Hodgkin, Huxley papers, and it’s certainly difficult to read other papers like even Clay’s and my own.
Part 11: The Book
Hille outlines the process, subject matter and motivations behind his 1984 book.
Kandel: You had written a couple of wonderful reviews. I don’t just mean the handbook one, you had done what, I forgot, the pharmacological reviews?
Hille: Almost every two years, I wrote a review. That’s right. The first one was in 1970 in Progress in Biophysics. Yes. So I had been writing reviews. So now I had about three motivations to tell you, and I had I sort of started in on one, but we got on another, and that is that I had written a lot of reviews, and they had taken a lot of time, and they were kind of long, and they often appeared in books that were published very slowly because they had other authors. And I thought, why don’t I just write a big review by myself. I could even get paid for it, maybe. And I would be in full control of what was in it and when it came out.
Kandel: Absolutely.
Hille: So that was one thing. And I thought, well you know, I’ve actually written a lot of reviews and so those would be the contents of quite a number of chapters already. I could almost take them and render it into the sequence and make my book. And in addition, I had taught an advanced course here in Seattle, where every year for ten weeks I reviewed some literature. One year it was calcium and calcium channels and calcium actions and all that, and I said in 1973, calcium is the ion of the future. And so I already had all the material I needed for a chapter on calcium channels underway. And I had done this for many other subjects, and each one could be a chapter in the book. And many of them were actually not published, because they were just the things I did in classes.
So there was that motivation, and then there was the motivation that we had to make sure that people didn’t rediscover stuff that was already known because it was hard for them to read about it, and so I had to make it more readable. And then I felt that biophysicists were really the people who had been doing all this work, and biophysics had somewhat run its course, by itself. I felt that if we just voltage clamped and could only measure open or closed, all these other states that we postulated or hypothesized, that we were not likely to learn everything you could know about channels, and we needed the help of biochemists, geneticists, pharmacologists, and all the other people.
Kandel: Structural biologists.
Hille: Structural biologists, eventually, fortunately. So I thought, well, you know what I could do is I could write a book where the chapters start very easy, and somebody could read it who was just a biologist. And then the chapters get harder and harder, but they do it so gradually that you don’t even know, you don’t even realize that it’s getting hard. So it won’t be like starting a book that’s hard, that says this field is too hard, I won’t do it. But you just keep going and getting fooled into reading the next chapter, not realizing that it’s getting harder and harder. So that was my strategy.
And I also felt that you had to be able to read the chapters in a finite time, so you had the satisfaction of saying, “I finished that chapter.” So I took every chapter as I wrote it, and I read it out loud. And if it took me more than 60 minutes to read out loud, I would say it’s too long and I’d have to make another chapter. So I actually delivered it orally. Any place that I slowed down while I was reading, I would mark in the margin, come back to and say there’s something wrong there. So those are different things about this book. It finally came out at the end of ’83 and had a copyright of ’84. I think that’s the book. The book went into the second edition in ’92, and now I’m writing the 3rd edition and I hope that it will be available in a year and a half or so.
Kandel: In the context of the book, it would be good if you brought in Rod MacKinnon and the structural biology and how the structure that he ultimately delineated was compatible with the work that you had done and Clay had done, and sort of provide a synthesis of our current understanding of at least potassium channels.
Hille: Okay, so if I say that it confirmed everything we said, I guess that would be kind of a proud, stuck up point of view.
Kandel: But it’s true.
Hille: But it actually was wonderfully reinforcing of what we had been doing.
Kandel: But maybe you could give specific details.
Hille: What Rod showed was that in this channel that he had crystallized, which came from a bacterium and doesn’t have the gates that open and close with a voltage dependence—it may in fact, in fact we know it opens and closes in response to something, but we’re not too sure what the right stimulus is yet. So it may even have some of the gates that we were looking for. The idea that Clay had started and I reinforced through our sodium channel work and our potassium channel work was that the major gate was on the inside, on the cytoplasmic side, and that, when it opened, revealed a big vestibule, which was large enough for large molecules like the local anesthetics and the quaternary tetraethylammonium ions and other things to go into and get stuck in, even when the gate is closed again. So there’s a big space, kind of a closet there where things can be put and the gate will be closed and they stay there.
We could measure the voltage dependence of the entry of these molecules or the number of molecules going into that place. And from my lab we had proposed that the voltage dependence comes because these molecules, which are charged like tetraethylammonium ion or the quaternary anesthetics, are driven by the electric field and pulled into the channel down a certain distance and the electrical distance, how much of the electric field they get pulled through, is easily seen by the voltage dependence of their binding. Now Clay had another interpretation. He said the voltage dependence came from potassium ions that were coming from the outside or the inside and striking the bound molecule and driving it on and off. And actually I think both are correct; they work together, as we showed in our long pore theory paper in ’77. So we had the idea, we found out that molecules would go pretty far from the cytoplasm towards the outside.
That was my view at least, and this means that the narrow place where the selectivity filter—which says this is a sodium ion or this is potassium ion and I like it or I don’t like it—that narrow place must be very far towards the outside of the cell, because these big molecules can go so far into the pore. And then we also, Clay and I and Bezanilla, said the potassium channel has a certain size, a diameter of 3 Angstroms, in order to just sort of fit a potassium ion, not let a cesium ion go through, and it’s coordinated probably by a bunch of oxygens, which make a dipole that it likes as substitutes for the water that it would normally have. And all of these elements were found in Rod MacKinnon’s structure. There was a narrow place, which had oxygens available to coordinate potassium ions. There were, in fact, potassium ions in the crystal, and they were sitting there, coordinated. There were several potassium ions as Hodgkin and Keynes had first proposed.
And then there was an enormous, surprisingly big—I can’t remember what Rod calls it—but there was a big cavity in the center of the membrane, half way through the pore, a water filled cavity in which there was also at least one potassium ion, maybe two. And so all of these things came from that. Rod has recently done theoretical work which argues, I think very nicely, that the cavity in the middle, the water-filled cavity is the trick that overcomes the electrostatic problem that an ion would have as it goes through a low dielectric lipid region of the membrane. If you make the ion go through a narrow pore without water all around it, the dielectric constant is very low and the ion doesn’t like to be there energetically. But if right in the middle of the membrane, where this problem is the very worst, you make a big puddle of water…
Kandel: A lake.
Hille: A lake. Yeah, it’s really quite happy. It’s not really out of water even though it’s right in the middle of the membrane, and then it can proceed through the rate-limiting barrier of the selectivity filter on the outside and all this electrostatic problem is taken care of. And Rod has made very nice calculations actually, with Benoit Roux and others, showing how that electrostatic stabilization works. I think those are really the features of Clay’s work and my work which come out. Subsequent work after ours, before Rod’s, like the work of Gary Yellen, identified residues that were amino acid residues on standard K channels that were facing the cytoplasmic regions, and ones that are facing the pore region…
Part 12: Roderick MacKinnon
Hille shares his impressions of and talks about his professional relationship with Dr. MacKinnon.
Kandel: Yeah, but some of that was done with Rod, right?
Hille: Some of that was done with Rod, yeah, that’s right. But particularly with cysteine scanning mutagenesis and things of that kind, residues were identified that had those properties. And on Rod’s structure, those groups were exactly where you might expect them to be in the crystal with exactly those kinds of positions as described. That was work that Clay and I didn’t do, but again another one of the wonderful confirmations that one gets from the structure.
Kandel: Did you actually interact much with Rod on the course of your…
Hille: Very little really. Rod was a student, a postdoc with Chris Miller, and Chris was a very good spokesperson—not that Rod isn’t a good spokesperson, but I sort of always heard Chris at Gordon Conference, you know, giving the stuff. For the second edition of my book I see that I liked Rod’s work a lot, and there are at least eight papers by Rod that are cited in the book. And I remember I sent chapters of the book around for people to read. I sent several chapters to Bruce Bean, and he pointed out that I had misspelled Rod’s name and he had gone down the hall to confirm, since Rod was there, that I had misspelled his name. So that sort of shows that I hadn’t really in 1991, hadn’t even gotten Rod’s spelling right. In 1991, maybe 1990, I heard him give a talk, and then I began reviewing some papers of his that impressed me tremendously…
Kandel: Yeah, he’s terrific.
Hille: And so around then is when I got to know of Rod and see him mostly at Biophysical Society meetings and the Gordon Conferences, and otherwise we haven’t had a lot of interactions. He’s been here several times to talk. I considered him at those times to be sort of a visionary preacher. He had a vision in his mind, which wasn’t actually fully specified from cause and effect in scientific arguments. He had kind of a conviction about certain things being the case, which he would then present to the audience almost in a mystical way, telling us of how wonderful it was—and he was right normally. I remembered him as a person who had this style—it certainly wasn’t a drab style of speaking.
Kandel: No, he was very engaging. What really sort of impressed me about him was this sort of Babe Ruth gesture of pointing to a set of fields and then hitting a home run, and that is he said, what needs to be done is we need to get the structure, and there’s no use my continuing to do biophysics. I’m just going to quit, find an environment in which I can have the kind of support I need in order to do this structure. And then to do this, despite the fact that a lot of professional structural biologists are trying it.
Hille: I mean here was Rod, who is training actually through Chris Miller, well his training largely was like the things like Clay Armstrong and I did, that is, electrophysiology, using those same kinds of things that we developed. And Chris Miller is in the biochemistry department and more biochemically inclined himself, so all those things probably impressed him.
Kandel: In fact, Chris was trying to get the structures, so this was what they did.
Hille: But that Rod would just throw it all away was really burning the bridges behind him. “I’m going to quit this and do the right thing.” We all knew it was the right thing. Nobody dared to do it. Nobody thought you could just quit if you weren’t already an expert in it and just pick it up and do it. He did it.
Kandel: It was wonderful.
Hille: Yes. So now maybe we should talk about things that we did more recently.
Kandel: Before we get on to that I just want to make sure that we have covered all the topics that you consider important. Up to the modulation, the more recent…
Hille: Yes. I think we have.
Kandel: Okay, good.
Part 13: More Recent Research
Hille describes his experiments demonstrating that a G protein without a diffusable messenger being involved can act on an ion channel and other work.
Hille: Let me say that after writing the first edition of my book, I sort of lost steam in a way. This was 1982, and I looked at what I had written, and there were 16 chapters at the time, and I had done a little bit of every chapter myself except for the Hodgkin Huxley, and I had done research work in those areas, and I had sort of exhausted the things that I felt I could solve in those areas. I think in a way, you work for a while in a field and have maybe a wonderful idea to begin with, and after you’ve worked through that idea, that is the best thing that you have, and you might as well move to a slightly different one, where you might have another wonderful idea. You shouldn’t just stay on the same one. You may not be so inspired, and it might just get monotonous. Now I know you haven’t worked that way. You worked on one idea and carried it forever and made it go in a more and more molecular way, and that’s absolutely fantastic.
But I don’t think that I can do that. I felt that I had sort of exhausted the way I could approach these biophysical questions. And I thought also, more or less as I had felt when I had started at Rockefeller, that actually this very biophysical research was less biological than I wanted to be and somehow was all tied in hypothetical structures and hypothetical states and curve fitting and arguments about that sort of thing. None of which was material and very little of which had to do with how an organism functions in its life and does its thing. So I was looking for other things to do, and that’s where ultimately, after thinking about a variety of things, we came into the G protein coupled receptor modulation of ion channels. And we actually came into that by pure serendipity. It was Paul Pfaffinger, who became a postdoc with you, and in this idea that I had mentioned before that we should tell the biochemists what a channel can do before they tell us.
I said, what’s the next channel that we have to do that hasn’t been cloned yet? And it was inward rectifiers, that very little was known about inward rectifiers. And now we know, for example, they’re blocked by spermidine and other very interesting things that physiologists might have discovered, but it wasn’t until the cloning that it really came out as the thing. So I said to Paul Pfaffinger, who was looking for a thesis problem, how about we look at inward rectifiers. And then I said, now okay, what system are we going to look at inward rectifiers in? So we looked at muscle. We looked at some RBL cells, which have an inward rectifier, and then I said, I know Neil Nathanson down the hall in pharmacology has chicken atrial cells, and we should also look at them just to see what their inward rectifier is before we decide which one you’re going to do this biophysics on.
And Neil was studying the effects of muscarinic agonists on changing the potassium flux in these atria, measured with tracer potassium, he told me, after I went down to his lab and said, can we look at some of these cells? And I said, hey, you know we could do that experiment better. We could do some electricity, and we could see these channels open for you when you add muscarinic agonists. And Neil was interested in G protein coupled receptors, about which I knew nothing. So this collaboration with Neil began with Paul just doing Neil Nathanson’s experiment better. And that was looking for potassium fluxes by looking at channels opening when you added acetylcholine analogs and asking if pertussis toxin blocks it, which was what Neil Nathanson was trying to do with his fluxes. And out of that came eventually the demonstration that a G protein in the pertussis toxin-sensitive Go/Gi family is involved in modulating, opening, the inward rectifier of potassium channels of heart.
Kandel: That was the first direct demonstration that a G protein without a diffusable messenger being involved could act on an ion channel.
Hille: That was the interesting issue. So first we just showed that you needed GTP. We showed that pertussis toxin blocks it, and then there was a literature which said from the past, an experiment done by Soejima and Noma, which was very like the experiment that Steve Seigelbaum had done in your lab, putting a patch pipette on a cell and saying, if you put the agonist in the bath, you don’t get modulation of the channels under the pipette, and if you put the agonist in the pipette, you do get modulation of the channels into the pipette. It’s the opposite from what Steve Siegelbaum found.
Kandel: Right.
Hille: And their conclusion was that there is no second messenger. So this was in the literature already that there was no second messenger. Now Bert Sakmann had been studying this channel, and basically he and Trautwein and Noma, who had been working together, treated this and more or less said it was like a nicotinic acetylcholine receptor that gated very slowly—because you had to put the agonist on the patch that has the channels in it, and you can’t put it somewhere else and have it generate a second messenger that goes to the channel. What we found was that there is a G protein in this process where there’s no second messenger. And I remember sitting at the computer on the very last day that we were going to send this paper to Nature, and Neil Nathason was behind me, and I was sitting at the keyboard, and Paul Pfaffinger was there and we were at this crux, the very last paragraph—what are you going to say? What is the best thing we can say? And I always learned that at this stage you were supposed to say the most bold and wonderful thing that you could say, and that would make the paper probably acceptable for Nature.
And so we debated what we could say. We said there wasn’t a second messenger. We said there was a G protein. And then we said, and then what? The options were to say that the G protein acts directly on the channel, or something lesser, and we chose the lesser route. We didn’t say that it acts directly on the channel. We said, “This is the first time that it has been shown that a G protein acts without using a cyclic nucleotide messenger.” That’s all we wrote. Without a second messenger. We debated both of them, and not having it written on a piece of paper and remembering Hodgkin’s statement, I wouldn’t want to say which side I had of the debate.
Kandel: If you’d been wrong, you would have been very pleased you took that position. So it was an appropriately cautious position.
Hille: If you read our paper carefully, we don’t actually say and we didn’t actually demonstrate that the G protein acts directly on the channel, but we did emphasize that there wasn’t a diffusable second messenger. This was ’85, and in ’87 then, both Neer, Logothetis and Clapham and Birnbaumer and Brown (Buzz Brown) began the arguments about, is it direct action? And then showed that if you put on G protein subunits you get actions. So we get credit of some kind, but we didn’t actually boldly come out and say what we didn’t demonstrate, but what we should have said.
Kandel: You set up the problem.
Hille: We did. Interestingly, then I told Paul Pfaffinger okay, now for your thesis—this wasn’t his thesis, this was a warmup—so what are we going to do? So we switched to another potassium channel, which also used muscarinic agonits, and that was the M current that Paul Adams and David Brown had discovered. And we thought, okay, this would just take a few minutes, and then we’ll have the answer to the M current because it will be the same. And it definitely wasn’t and even today, our laboratory is still studying what second messenger mediates this process, and we don’t know the answer.
And then we started working on calcium channels, whose opening is depressed by G proteins instead of their being opened by G proteins or by receptor action. So as Cathy Dunlap and Gerry Fischbach showed, the current carried by certain calcium channels can be decreased by many agonists, norepinephrine, acetylcholine being among them. And they proposed correctly that this might be an explanation for pre-synaptic inhibition. And we began to study that and characterized it in many ways. Only recently, or maybe ’95 I guess, we showed together with Bill Catterall’s lab that the G protein beta gamma subunits act directly on the channel in that case too. So we sort of came back to this direct G protein interaction. The potassium channel, I guess, was the first case where the beta gamma subunits…
Kandel: Yes, that was the other interesting thing to come out of it, and that is that the Clapham and Neer argument was a correct one, and it was the first demonstration of a beta gamma subunit acting as an effector.
Hille: Yeah, and it was a clear one. And the calcium channel becomes another and there are now quite a number of effectors. Probably more effectors for the G protein beta gamma subunits than the alpha subunit are presently known. Okay, well that’s really the kind of problems that we’re still working on today. How do you modulate ion channels? We’ve also worked a lot on calcium dynamics and other cell biological questions, which probably we shouldn’t get into and aren’t related to the Lasker Award part. I think we’re done, Eric.
Part 14: Perspective On Ion Channels Field
Hille talks about how the field of ion channels has changed from its early beginnings to today.
Kandel: I think that’s wonderful. Give me just sort of a few perspectives on your career, of how the field of ion channels has changed from our early conception to now.
Hille: Okay, changes, there are several. One is, at that time people didn’t know they existed. They didn’t know there were pores and so forth. Every time you began to lecture about doing the kinds of experiments that we did you’d have to spend ten minutes telling people that there is a sodium potassium pump and you’re not talking about that kind of molecule that uses ATP, you’re talking about a different molecule. Nobody knew about it. In fact, nobody cared about it.
So when I began my thesis work, there were fewer than 20 papers to read altogether on voltage clamping, and probably three or four papers appeared each year. And now if you search on Medline, 5,000 papers appear every year, and you can publish it in Nature and Science and Cell and Neuron. It’s a very highly competitive field in which the molecules, which we weren’t even aware of at the time, have been cloned, and we have the proteins. We’re beginning to get the crystal structure of the first ones. We have molecular diseases so that quite a number of human inherited diseases have been shown to have mutations in ion channels of which the most famous is CFTR, cystic fibrosis transmembrane regulator, but many in sodium channels and calcium channels and potassium channels. So the field has grown by several orders of magnitude.
In 1980, Neher and Sakmann and their colleagues published the patch clamp method, and this changed completely the approach. You no longer had to stick wires inside cells and so forth, and furthermore Fred Sigworth and Erwin Neher designed an amplifier, which then went on the market. And this was the first time that you could buy equipment off the rack that allowed you to do experiments with voltage clamps. And since then, about 20,000 of those boxes have been sold by the various groups that now supply them, all of whom designed them because of some electrophysiologist who made a design, and then it was licensed.
I don’t think anybody has designed a patch clamp who wasn’t already a practicing electrophysiologist. So instead of having to make your own amplifiers which I did myself at that time, and make your own computer interfaces, in fact not use a computer at all for most people, now you buy the computer program. You can buy the computer for a very low cost and you buy the amplifier and you buy the stimulator. So basically you just ask for $50,000, and you get a set up completely made, and nobody who works with you has to know how it works. You get on the phone and a service person can guide you through whether you’re supposed to send it back or not. So that is sort of the mechanical side.
Intellectually, I think the quality of work has been high all the time. It’s very high now and it was high from the beginning. Certainly Hodgkin and Huxley take full responsibility for setting the level where the bar is. It’s very high. You have to be good at quantitative thinking and good at logic and so, although we have lots more people, the quality has stayed about the same. But the kinds of things you can do is enormously expanded. Instead of just looking at electrical currents you can see fluorescence changes of molecules. You can test individual amino acids. You can test protein-protein interactions all these things make it just wonderful. So I would say it’s very fulfilling to see the growth of interest. The fact that we had to previously kind of push uphill to even get the audience to open their eyes, and now often people assume that it must be interesting, so they come with bright faces and open eyes and listen.
Kandel: Well, it’s the central theme of neurobiology, and what I really have admired about your work and Clay’s is that essentially you took what was a black box approach. I mean, without seeing the structure you were able to characterize it so satisfactorily that it provided an excellent guide for the cloners and the structural biology.
Hille: I guess it’s really the power of biophysics.
Kandel: That’s right the power of biophysics.
Hille: Or of voltage clamps.
Kandel: And good thinking about it. Yeah. I don’t think it’s just the voltage clamp, and I don’t think the methodology, because you guys really didn’t introduce that much methodologically in terms of modifying the voltage clamp.
Hille: Yes, that’s right.
Kandel: I mean you used the transistorized model.
Hille: And think of how much Hodgkin and Huxley found out just from one set of papers.
Kandel: That’s right. Just a few squid giant axons, as Zeki would say.
Hille: They set the stage, and Clay and I continued with a much more molecular desire.
Kandel: That’s right. That’s what you introduced, the molecular feel of it.
Hille: Though we always had in mind that we were going to figure out what the molecule looked like. Very interesting to me and I don’t have any explanation, that once we could figure out what the molecule looked like at the point of view of cloning, I stopped doing the work.
Kandel: Well, you had solved what was for you the important aspects of the problem.
Hille: Well, I solved what I felt I was good at solving. And so I went to something else.
Kandel: Modulation in the mind.
Hille: There we go. I feel good.
Kandel: I feel very good. I feel you have done a wonderful job in describing your work, and I think it’s terrific work, which should give you a lot of satisfaction.
Hille: Thank you for your generosity in taking us through this.
Kandel: Oh, this is lots of fun. This is very exciting for me.
Key Publications by Bertil Hille
Hille, B. (1967) The selective inhibition of delayed potassium currents in nerve by tetraethylammonium ion. J. Gen. Physiol. 50: 1287–1302.
Hille, B. (1971) The permeability of the sodium channel to organic cations in myelinated nerve. J. Gen. Physiol. 58: 599–619.
Hille, B. (1977) Local anesthetics: Hydrophilic and hydrophobic pathways for the drug-receptor reaction. J. Gen. Physiol. 69: 497–515.
Dwyer, T.M., Adams, D.J., and Hille, B. (1980) The permeability of endplate channel to organic cations in frog muscle. J. Gen. Physiol. 75: 469–492.
Pfaffinger, P.J., Martin, J.M., Hunter, D.D., Nathanson, N.M., and Hille, B. (1985) GTP-binding proteins couple cardiac muscarinic receptors to a K channel. Nature 317: 536–538.
Herlitze, S., Garcia, D.E., Mackie, K., Hille, B., Scheuer, T., and Catterall, W.A. (1996) Modulation of Ca 2+ channels by G protein beta-gamma subunits. Nature 380: 258–262.
Roderick MacKinnon interviewed by Dr. Miller
Part 1: Early Education and Medical School
Dr. MacKinnon describes his upbringing in suburban Boston, his early interest in science and his earliest years in Dr. Miller’s lab at Brandeis. He also discusses his decision to go to medical school and its impact.
Miller: So why don’t you just tell a little bit about your personal background and sort of the trajectory by which you arrived at the place you are at now.
MacKinnon: An unlikely trajectory. I was born in Burlington, Massachusetts. I’m a Massachusetts boy. The middle of a family of seven children and grew up in Burlington, Massachusetts. Went to the public school there, and my parents moved to Cohasset when I was in high school, in ninth grade, (they) moved to the South Shore of Boston. So I’m a Boston suburbanite, all my life. And related to science, you know my parents are not scientists, and I, in fact, didn’t know any scientists when I was young, but I always loved science. They were my favorite courses in school. And I guess you could say that it became clear that something wasn’t quite right when in junior high I wanted to go to summer school because it was a science enrichment course, and I got to have a microscope there, and I could take the microscope home for the summer. I used to like to go around and look at pond water and blades of grass, and I was thoroughly amused by these things.
I think I was a very typical average little kid in most respects. But when I really did get turned on to science is when I went to college, to Brandeis University. And there I went from public schools in suburban Boston to Brandeis University, and that was a real eye opening experience for me. There were a lot of my peers there, my classmates, who were from different backgrounds and I just… It was a real good time. It was from 1974 to 1978. Brandeis was a wonderful place to be then. I had a lot of fun, and it was a real intellectual experience, and my exposure to classroom science was very good there. But in particular, Chris, as you well know, I came to your lab and worked on calcium pumping in the sarcoplasmic reticulum. I was the third member of your lab, you being the first, Gribet your dog the second, and I was the next. When you had just set it up. And it was a special time. I have a lot of emotions attached to that, actually, in the little lab.
Miller: We both do.
MacKinnon: It was a very bright lab, big windows. The sun would pour in, and it was just a happy place to be. It was a wonderful time. I ended up going to medical school.
Miller: Against my strong advice, if you remember.
MacKinnon: I remember very well. In fact, I think the real reason I went to medical school was not because I was ever pushed to do that, but I was naive enough to think that the practice of medicine was close to the carrying out of scientific experiments, and I learned that it’s quite different. You use a different part of your brain in solving problems in medicine than in solving problems in a more analytical kind of way. Here’s the puzzle, can you solve it? That’s what I loved about science and do love about science. But I think I went to medical school because that’s what everybody seemed to be doing, and I did think it was a lot like laboratory science. It took me a long time to figure out that it’s very different. It’s very special in its own way. I don’t have any regrets, but I know that when I came back to you eight years later and said, “You know I’ve been thinking about what you said, and that is that I should get a PhD” You know it’s a funny story, and it’s absolutely true. I came back eight years later and said, “You know, you were right.” And, fortunately, because then I could get a postdoc.
Miller: Yeah, you didn’t realize that. You thought you had to go back to graduate school. And I said, “You dummy, you don’t have to get a doctorate, you already have a doctorate.”
MacKinnon: I had completed medical school in four years and three years of residency with the plans to practice medicine. And although I felt like I was getting old at the time, close to 30 years old, I just thought about, “Gee, when I’m much older than this, I’ll look back and say, gee, that was actually young. So just go for it.” So I thought I should go to graduate school and get a PhD, and you advised me that I wouldn’t need to do that. So that’s when I came to do the postdoc at Brandeis with you, in the same lab, and started working on potassium channels.
Miller: I think your medical education actually gave you a tremendous kind of insight that allowed you to do some of this work in a way that was very different than if you had gone through the sort of way that I had suggested.
MacKinnon: Well, I think it did in the following way: One of the things that I did in medical school… Well what it did, it taught me to teach myself very well. A little background to that is that while I was going through medical training, I felt I had a need to exercise my mind in a certain way, and I applied mathematics. It became a hobby of mine, and I kept studying those things, and that was good for me. And then when I came to your lab, I had a real sense that I was way behind. And so I read and read and studied and, you know, I’m very diligent about these things. I have stacks of notebooks where I derived things, and I just wanted to make sure I understood things completely. In a way, what that did for me is it taught me to be very independent by just going to learn something. And I think if maybe had I gone to get a PhD, I would have… I don’t know what would have happened, but this way I certainly know I learned to fearlessly pick up books, go get books and learn a subject, and that certainly came in handy as time went on. That sort of approach.
Miller: Yeah.
A suggestion by Miller that MacKinnon work on a scorpion toxin yields many wonderful results. When MacKinnon set up his own lab at Harvard, he says, “the most exciting points that stand out in my mind are the experiments showing that potassium channels had to be tetramers.”
Miller: Well, why don’t you just say a little bit about when you started your own lab, and then what kind of research you started out doing and how then, what made you make this big essentially almost a mythic heroic change into crystallography. I think you are the only person in the field of ion channels who did this. Who just dropped the standard way of studying ion channels that we’ve all been doing and just said, “No, I am going to now remake myself.” Tell the story a little bit.
MacKinnon: I’ll tell the story, but actually the story begins, in thinking about it right now, a little before the transition from mutagenesis analysis that we were all doing. I think the story begins when I came to your lab. Among the things I read, I read the classics of Hodgkin and Huxley. I read the work of Bertil Hille and Clay Armstrong, the sort of electrophysiologist view of ion channel proteins. This field, its background is very different than the rest of biochemistry. For example, the people who taught us how enzymes work, they had their background in protein biochemistry. So mechanistic enzymologists knew all about proteins, whereas, for historical reasons, the study of ion channels compared to enzymes had a very different background. People who studied ion channels were people who knew about resistors and capacitors and amplifiers—very different kinds of people—and less about proteins. So the techniques were completely different. The study of ion channels for so many years was almost like a parallel universe.
Miller: Different language, different…
MacKinnon: A totally different language, you know, and when people from the protein world start hearing about capacitance, I think that you know that is something that sounds strange. And likewise when people from the electrophysiology world would start to hear about hydrogen bonding, it would sound equally strange to them. I think the whole field was quite separate. But when I came to your lab, it was very clear to me that in this environment of Brandeis biochemistry, you thought about ion channels the way Jencks and Abeles thought about enzymes. And it’s really true. So my introduction to the field is, although I was reading the classic electrophysiology text, I was also being immersed in this way of thinking about ion channels as proteins.
And I remember going over Michaelis-Menten kinetics and at the same time thinking about ion channels in the same way, because your lab was carrying out experiments on sodium channels and potassium channels and analyzing it in very much the same way. You know, trying to decompose free energies of transition states into enthalpy and entropy double daggers, and this was thinking of ion channels as proteins. And so that’s where I got my beginning, and so that’s where I think for me the story begins, for looking at ion channels like proteins.
You know, a lot of beautiful happenstance occurred because you suggested I work on a project with this scorpion toxin. You had discovered it. You found out it blocked potassium channels, but wanted to know more about the mechanism. And taught me quickly how to use the bilayer and said, study this. And that was beautiful because in a way it was a very, very simple problem. A very concrete question and good for my learning, and to start thinking about binding energy, and having a picture right from the beginning in my mind of what a channel must look like, and the toxin coming on to it. It was just a perfect project to be working on, especially when around the same time, the first potassium channel gene was cloned. The shaker gene.
I mean this is a nice coincidence of events, because then we discovered, of course, that the shaker gene channel was blocked by the scorpion toxins and immediately had a tool to go at and ask where these amino acids go in the three-dimensional structure. So in a sense, it all begins there with thinking about the three-dimensional structure of the potassium channel. And you know, one thing led to the next.
Before I left your lab, we had found the first mutations on the shaker channel that affected the toxin binding and started to have—it was a very exciting result—the first nibbling of information of whether something is on the outside of the membrane or the inside of the membrane. And is it near what we imagine the center of the channel is, where the pore is, or is it far out on the side? So that’s where I think it all began. And then in going in 1989 to my own lab at Harvard continuing on the problem. That couple of years was a great chapter, I think, in all of ion channel biophysics for potassium channels. What went on…
Miller: So much was happening.
MacKinnon: So much happened. What went on in my lab, in your lab, in Gary Yellen’s lab, in Rick Aldrich’s lab and others. It was just over a period of a few years where mutagenesis was used, and we discovered a lot of things. It was very exciting. I mean, I would say from my own efforts that the most exciting points that stand out in my mind are the experiments showing that potassium channels had to be tetramers, although that was a result that everybody more or less expected. It was using the binomial statistics and the toxin to show that it absolutely had to be. It was a thrilling set of experiments for me. It gave me this picture that there definitely had to be four subunits symmetrically arranged in a ring, and then the mutagenesis showing the pore loop. Many labs contributed to that, but because of the toxin work that started in your lab and then continued in my lab, we really found this pore loop.
Miller: That was a totally new idea when you found it. The idea that the pore would be lined by this loop of sequence rather than a transmembrane helix.
MacKinnon: That’s right.
Miller: Everybody was looking at, where is the transmembrane helix that will line the pore?
MacKinnon: That’s right. We always imagined there would be cylinders going around the central pore and residues on the cylinder, which if you then looked at this linear stretch of amino acids, they would, we assumed, be scattered throughout the protein. But actually that was a very surprising result. And I think an important result for me psychologically—the realization that you had a barrel with the loops reaching in, and what determined selectivity was a linear stretch of the amino acids. Because I always just assumed when you put the polypeptide chain up, put it together, the residues that would be important would be in one region in the structure, but when you unfolded the whole thing, they would be spread out. But in fact, that was not how it was.
Miller: As you have in enzymes.
Part 3: Burning Bridges
MacKinnon elaborates on his work on potassium channels and speaks candidly about his momentous decision to leave Harvard for The Rockefeller University.
MacKinnon: As you have in most proteins, right. But in the potassium channel and now we know this is going to be true for calcium and sodium channels and some others. It seems there are determinants in a very small stretch of amino acids. And the reason I said that was important psychologically is because I thought, “Gee, it begs the question, what is special about these amino acids and what are they doing? Why is a linear stretch of amino acids making the selectivity filter of a potassium channel?” We could only take the mutagenesis as far as knowing which amino acids were responsible for selectivity.
But beyond mutagenesis, we couldn’t answer the question, why do they do what they do? And that was the real motivation for me then to say, “Okay I have to adopt a new set of techniques.” And that’s after I was at Harvard for about four or five years that I started thinking this way. And the other thing was, the cloning efforts on potassium channels, cloning efforts throughout the world, showed that potassium channels from every part of life contained the conserved sequence. So then not only did we know that it was a linear stretch of amino acids in a small region that are important, but that nature seemed to be happily settled on one potassium selectivity filter. That begged the question even more. What’s it doing and why is it the selectivity filter?
So I thought about different structural techniques and decided that x-ray crystallography would be the technique of choice. So what I did is I, well, I got books. And as I said earlier on in this interview, that was one of the things about my history coming from medicine and then feeling that I had to catch up, I learned to learn on my own very well. That helped when it came to trying to adopt a new technique. And the way I did it was I read a lot of books, and I went and talked to a lot of colleagues who were experts to learn as much as I could. First with purifying proteins and crystallization. So what I did was I started purifying some soluble proteins and trying to crystallize them. One was the scorpion toxin, and I eventually got crystals of that, but they were twinned. And that was around the time we started getting our first crystals of the potassium channel, so I set the toxin aside. But then also, the PDZ domain, that was a good etude, a soluble protein that I could purify and crystallize. Meanwhile, at Harvard a lot of people in the lab thought I was losing my mind because they were, I mean…
Miller: I think a lot of people in the field thought you were losing your mind, Rod.
MacKinnon: Well, perhaps I was. Yeah. I had a lab filled with some real talented postdocs and students and they were carrying on the mutagenesis work and analysis with my sort of guidance, but I spent all my time, most of my personal time, learning about chromatography, purification, crystallization and the fundamentals of x-ray crystallography. And then I ended up moving from Harvard to Rockefeller and that was a complicated, I would say a very complicated time and decision in my life. I guess if I think back on it and decide why did I move at the time, I think it was mostly because I really wanted to throw myself off the deep end. I didn’t want to be distracted.
Miller: When you moved you were essentially burning bridges, you felt.
MacKinnon: Yeah.
Miller: What exactly were the bridges?
MacKinnon: The bridges were, I had a lab full of very talented people and the people who were applying to come to my lab wanted to learn—reasonably so—wanted to learn what I was good at.
Miller: Classical mutagenesis…
MacKinnon: Classical mutagenesis and electrophysiological analysis of mutated ion channels. And we did learn a lot from that. But I think that’s what it was. Nobody wanted to come work with me to do x-ray crystallography. Young scientists in channels don’t want to go do crystallography, particularly with somebody who has no record of it. And those in crystallography didn’t want to go study membrane proteins by and large, in particular with somebody who had no track record.
So I think the bridges I was burning were those… I was taking away my security blanket of being able to fall back on the techniques that worked for me, and I knew if I just jumped out there and went for this, it was a do or die situation. And when I moved it was just me, one postdoc who had just joined the lab, Declan Doyle, and my wife, Alice. She really felt bad for me and decided that she would work for me, having trained as a chemist. So when we got to Rockefeller it was just the three of us, and that was scary. I was embarrassed to have people visit me, because they gave me a nice lab. I thought people would think, “My God, you know you don’t have anybody in your lab. Why do you have this space?”
Miller: I mean it’s true that most, nearly all—I would say all in my knowledge except for you—people who start new directions in their labs, and of course starting new directions is something that every scientist has to keep doing, but the way to do it, the conventional way we all do it is to do it with, just what you said, with a safety valve where most of your effort is still being spent on things you know how to do as you try to learn something you don’t know how to do. But you didn’t do that. You really just went in to do the things you didn’t know how to do. You went through quite a few years without publishing anything, and I guess the question is—I really don’t know if you want to talk about this—how did you feel as you stepped away from Harvard into this new lab at Rockefeller, and you really were jumping off the deep end, as you said. How did it feel?
MacKinnon: I was scared. I was really scared, actually. Do you remember, with this decision I came to you, and I said I really needed to talk. In fact, we got together and we walked down to a little pond in Needham.
Miller: I remember it very well.
MacKinnon: And I told you what I wanted to do.
Miller: The second time I’ve given you advice that was worthless.
MacKinnon: Well, you know you thought it was a great idea, but you were more reasonable in thinking why don’t you hang on to some of what you do. It will take a long time. But I guess that’s just part of my nature, of sort of just going. You know I’m very focused. I think I can only do things if I can focus hard and really brood on a problem and just have no other distractions. And that’s what I guess I knew inside, what I had to do to make a go of it. It was frightening.
Part 4: Ion Channels Overview
MacKinnon recounts the amusing “white board” story from his days at Harvard and reflects on the overall state of ion channel research.
Miller: Just along those lines. That was one of those things ever since I knew you as a young undergraduate and as a postdoc, but I was particularly amazed at it when you started your own lab at Harvard. It seemed to me you had a real, I don’t know if talent is the word, but an amazing capacity to not be distracted by all the things that distract us. I think that is something that you’ve held onto. I remember in your early years at Harvard, I was amazed at how you didn’t get distracted—and I think you alienated a lot of people in your department—because of your ability to just focus on your work.
MacKinnon: Yes, that’s right.
Miller: I remember an incident with a white board.
MacKinnon: Well, I remember that.
Miller: I don’t know if you want to talk about it. It’s a good story.
MacKinnon: You mean the white board leaning on my wall in the office and the chairman really not finding the appearance of my office appropriate. That’s right. The white boards were put there because it was a very good way to organize things and work out little problems, but it looked, I guess it didn’t…
Miller: They weren’t mounted on the wall.
MacKinnon: They weren’t mounted on the wall, and I most efficiently used the office space by stacking long white boards that should be hung horizontally, vertically so that I could make the whole wall white boards. And I guess I can understand why the chairman would feel that visitors to the department would wonder, what do they do to young junior faculty at Harvard? They treat them very well, but I was just very focused and very efficient about things. I seldom went to the faculty meetings and the department administrator used to come down. She liked me very much, but was very worried about me and my future, because I wasn’t doing everything that I should. You’re right, I guess I’m very single minded when trying to solve a problem.
Miller: Let me ask you about the structure itself, and right now we are still just a little more than one year downstream from what I would call “first light” in ion channels—of actually seeing what the pore of one of these selective ion channel proteins looks like. What would you say are the things that, to your mind, are the most important things we’ve all, as a field learned, from this? At this early stage in what is now the structural era of ion channels?
MacKinnon: I think one of the lessons, and that’s sort of a methodological lesson, is that the field has done very well in that many of the predictions that were made were very, very good in terms of the general organization of channels, so that the techniques being used by electrophysiologists to study these channel proteins are teaching us a lot. That’s on the methodological side, but in terms of the structure and what does it tell us new, I think it answered a few questions and then it raised as many. And I think the kind of question that it answered, and maybe the one that I was most struck by, was how the architecture solved this dielectric barrier problem, actually.
You know the idea that everybody knew for ages that a very basic problem in biology is that because ions, because of their electric charge, they have an electric field around them, and therefore, they are repelled by the low dielectric, oily cell membrane. So nature had to concoct some mechanism for lowering that repulsion called the dielectric barrier. When we saw the first electron density maps, we could see the main helices. But with averaging, the maps were quite good, and we could immediately see that at the center—or half way along the pore—there was a cavity in the middle that was big enough to hold quite a bit of water. And that would be right at the center of the membrane. And then there were four alpha helices pointing their C termini directly at the center of the cavity.
And you know, having studied electrostatics a lot, that aspect of the structure spoke to me. And that was nature’s solution to this dielectric barrier problem. That is something I think that the structure simply answered. The use of the pore loop in its organization of amino acids to get the main chain atoms pointed at the selectivity filter was also beautiful. We still need to see this at better resolution to better define the chemistry. But what’s very clear is that this is a main chain carbonyl oxygen selectivity filter, and all held together very beautifully by this structure. So these aspects to me, the structure simply answered.
Miller: What is the question that it answered in the ligation of the ions going through? What was the main mechanistic puzzle about what these channels were doing? That I think your structure really…
MacKinnon: Yes. Well, very early on the view was, do ions go through the pore hydrated or unhydrated? But I think you know that was very nicely demonstrated through electrophysiology experiments, and some of your own, that the potassium ion is mostly dehydrated. So we knew it was dehydrated, but the question is, how is it coordinated? What atoms of the protein coordinate it? And in fact, there was a hypothesis put forth by me: Could it be, aromatic rings? We could see from mutagenesis a few years ago that the tetraethylammonium ion on the outside was coordinated by a phenylalanine or tyrosine, or a group of four of them in a ring. Because the pore loop amino acids always had some quite conserved aromatic amino acids, we wondered whether metal cations could be coordinated that way as well.
Metal ions could be coordinated by the p orbitals of aromatic rings. And in fact many theoreticians and chemists went and worked on that idea. And still there are some who really like that idea and are quite attracted to it. This structure dispelled it. It doesn’t work that way.
In fact, I think some of the questions in my mind that are raised by the structure that are just fascinating are—you know, what it did with the aromatics was actually they are in a cuff around the selectivity filter, pointed away from the selectivity filter, and they form that beautiful plane—and why does nature seem to want aromatics there? And in fact, they are packed in a very unusual way for aromatics. Instead of being herringbone packing with a sort of partial positive edge of a ring against the partial negative face of an adjacent ring, in fact, they’re more like edge on edge with funny tilts. But there’s a beautiful cuff around there, and I think it has something to do with the compliance or sort of flexibility or lack of flexibility of these rings that ensure that the selectivity filter is quite geometrically constrained to do selectivity. But the fundamental question about is it oxygen carbonyls or aromatics, was certainly answered by the structure, very, very beautifully.
You know fascinating questions that I think about on the structure are, why is this inner wall of the cavity and inner pore, we call it, the part below the selectivity filter that is near the inside, why is that hydrophobic in nature? We proposed that it tends to be inert so that ions won’t stick to it, and I think that’s right. But I think there’s more to it. I actually think it has to do with, the way it structures the water in there, and we won’t understand that until we have a higher resolution structure that nicely defines the water—and understand more about water against hydrophobic surfaces. This is speculation, but I find it fascinating.
Another aspect of the structure is that on the selectivity filter… You know the fact that the three ions are in the queue, this was a result anticipated for more than 40 years from the work of Hodgkin and Keynes, and then from the work by you and Jacques Neyton using barium in the calcium activated potassium channel. We knew they’d be in a queue. But then to see them. I think it really said that there must be mutual repulsion in there, as you and Jacques had proposed. To see that and to see how close the ions really are, I think that actually the selectivity filter is going to be an interesting chemical device where the ions not only interact with each other energetically through Coulombs law, but I think they’re going to interact energetically through the structure. That is, binding at one position at the end of the selectivity filter perturbs the structure enough to affect the affinity of binding at the other end. I think that’s going to be key to understanding the energetic interaction between the ions and why you get near diffusion-limited throughput with such high selectivity. You know that’s probably the question that drives me now the most. I really want to see that filter at high enough resolution, and probably it is going to require dynamical studies using spectroscopy, to see how this thing moves with different ions in it.
Part 5: Psychological Wall
MacKinnon and Miller discuss what they see as a psychological wall that is crumbling with the emergence of structures from the works of MacKinnon and others. MacKinnon also raises his concerns about NIH funding and its support for structural genomics.
Miller: Let me ask you something about membrane protein crystallography right now. It seems to me as kind of an outsider having been looking in at this field for some long time, in a way a lot of feeling like a kid looking into the candy shop window, it feels like that last year with your structure emerging and Doug Rees’ structure of the mechano-sensitive channel emerging with Senyon Choe’s structure of the T1 domain, these ion channel structures suddenly appearing, bursting forth all around the same time, it seems to me that there is a psychological barrier almost that has been prevailing in the field of ion channel studies in general. Everybody who wanted to know about mechanisms of ion channels for the last 20 years has been thinking, “Oh, we need a structure, but we can never get a structure.” And there are all these horrible reasons why it’s virtually impossible to crystallize membrane proteins. Very few of them have been crystallized, and so on. And I think everybody, except a very small number of people, were simply frightened by this. But now in the wake of your structure and these others that then followed it, it seems to me that there is this a psychological wall that’s beginning to crumble. Do you think that that is just a fantasy, or do you think that actually there’s going to be some movement now in the future, that this acceleration is going to happen?
MacKinnon: I think it’s absolutely true. I think there is a psychological wall that’s crumbling. If you think about it, the small number of membrane proteins that were solved, were solved in a very small number of labs, who did a couple, and they were very well known for that. And you know, it is a hard problem. It’s not that the problem is trivial, but I think that there is a psychological barrier coming down, in that there will be new methods for getting this done, as well, that we’ll see in the next few years. I don’t know what brings about that change, I mean practically speaking. I’m sure that if my little lab with little experience could actually do this then that’s a good reason for people to believe, “Gee, these are solvable problems.” You don’t have to be a detergent biochemist for 30 years to be able to do this problem. And I think that with a larger number of people doing it, we’ll see more and more of these structure coming over the next few years.
Miller: From where you sit, do you see more young crystallographers emerging from their postdocs beginning to be willing to undertake membrane proteins or …
MacKinnon: They’re not pouring in, actually. They’re not pouring in.
Miller: It’s still very risky, I would think.
MacKinnon: No, they’re not pouring in. I think they view it as quite risky. Yeah, so the number of labs doing membrane protein crystallography is still quite small, and it is still risky, and I have colleagues who are working very hard and are worried. And rightly so. Because the problems are hard. And I have to emphasize that part of the difficulty is the problems are harder than a four-year cycle of an NIH grant. They are harder than that, and it has to be recognized.
Miller: And most of those problems were solved in Europe where the scientists there were not under the four-year, five-year cycle.
MacKinnon: In my view, there is a very good reason why those problems have been solved outside of the United States, right, because I think that people couldn’t take the risk here. I guess I left one reality out in thinking about it. I talked about the reasoning for moving was creating a do or die situation, but also you know, I had to fund this effort somehow, to be plain and blunt about it. You know, I got a start up package that helped me initiate this effort, and then I was fortunate enough to become a member of the Howard Hughes Medical Institute. But yeah, I think it’s very important that it be recognized that these problems take more than four years. They are solvable, but they take more than four years, and people have to be supported for longer than that.
Miller: Yeah, and of course that’s a difficult choice when you have limited funding, is how do you know where the likely support, where the likely payoff is going to be, and so on. Do you see that there’s a way to work this out within the confines of the NIH funding system?
MacKinnon: Oh, I’m sure there is, but a special effort has to be made. And I don’t mean to upset colleagues to hear what I have to say, but in some ways I’m nervous about structural genomics and the NIH seeming to want to go ahead and support this. Solving membrane proteins, aside from what a few people may say or think, in my opinion, solving membrane proteins won’t be served by structural genomics efforts. These kinds of very difficult problems, I think, need to be categorized specially by the NIH.
Miller: Why exactly do you think that structural genomics is not going to help with membranes…
MacKinnon: Because if the goal of structural genomics is to identify many folds very quickly, people are not going to be turning to membrane proteins to do that.
Miller: Do you think there are going to be a lot of new folds in there that haven’t been seen?
MacKinnon: I’m sure there will be some… In membrane proteins?
Miller: Yes.
MacKinnon: I don’t think membrane proteins are going to be fundamentally different than soluble proteins in that regard, in their folds. Yes, there’ll be new folds simply because the proteins had to solve different tasks and they tend to be living in, you know, a very constrained world of a planar lipid membrane. But beyond that, I just think the difficulty of membrane proteins makes this massive approach of quickly solving structures—membrane proteins are not good subjects for that, that’s all. Just for a very practical reason. Yeah, and I think that it’s still going to be for a while that the people solving membrane proteins, compared to people doing structural genomics, are going to be the artisans, who care very much about a particular protein or a particular class of proteins and are willing to risk their careers on solving them.
Part 6: Further Reflections
MacKinnon offers reasons why “Aunt Gertrude,” i.e., the taxpayer should care about scientific research and has this advice for young scientists: Take a risk.
Miller: I wonder if you have a speculation about a fact that I’ve been struck by over the last years seeing membrane protein structures emerge. And that is that every single membrane protein structure that has been solved by crystallography is a prokaryotic membrane, is from a prokaryotic organism or at least from an honorary prokaryotic like a fluroplaster.
MacKinnon: Yes, there are a few exceptions, but almost exclusively that’s true. I don’t know. Well, one point is, well there’s beefheart.
Miller: That’s mitochondria. That’s an honorary prokaryotic.
MacKinnon: Okay.
Miller: I think if you actually add them up there’s nothing from a…
MacKinnon: I think there’s a…
Miller: Oh yes.
MacKinnon: One that’s plugged in.
Miller: That’s right. There are some lipases, that’s true.
MacKinnon: There are a few exceptions, but you’re right. I think that’s largely because of availability of protein, not because of any special stability. Yeah, I think so. There may be a little more stability, but I don’t know the answer to that, and I don’t think that it is going to be that much more difficult, once the cell biology problem is overcome.
Miller: Of, you mean, making a large amount of a crystallographic quantity of protein.
MacKinnon: Right. There’s a light harvesting complex. There’s a plant light harvesting complex, as well.
Miller: Chloroplast.
MacKinnon: Okay. Yes okay, I should have listened very carefully to you (laughs). Yeah, you’re right.
Miller: But certainly the stability of things like KCSA potassium channel, of the mechano-sensitive channel, and the porins, certainly those had to have contributed a lot.
MacKinnon: Thermodynamic stability, I think, is very important in being able to solve the problem. And you know for some of these, it might mean we’ll have to develop some genetic screens to screen for thermodynamically more stable versions of what we want in order to really solve the problem. It’s going to require a lot of resourcefulness on the part of us all to solve some of these problems, but it’ll keep us out of trouble.
Miller: One of the hardest questions, I don’t have to face the question very often, but when I have, it’s one I always trip up on, and I don’t know, I never come out with a very good response.
MacKinnon: Thank you for asking (chuckles).
Miller: So the question is, why should Aunt Gertrude care about this kind of research? Or instead of Aunt Gertrude, let’s say why should the taxpayer support this stuff?
MacKinnon: It’s a very good question.
Miller: I mean it’s not a trivial question, but a very important one. Why should the taxpayers care whether you are having a good time seeing three ions in a row inside of this pore?
MacKinnon: It’s a very important question, and you know, there’s no question in my mind that ion channels are going to be very important targets for pharmaceuticals in the future. They already are in some cases. But partly, it’s limited I think largely because of this parallel history of channels being studied in an electrophysiological way, rather than a biochemical way, and that’s because channels are scarce. We couldn’t purify a lot of them. But I think our ability to purify them, to understand their structures, how they work, is going to open new ways to treat diseases like epilepsy, cardiac arrhythmia, hypertension, asthma. I’m convinced this is going to happen.
And one might ask, well, how many diseases are related to the abnormal ion channel? And the answer to that is, well, I really don’t know right now, but I do know that there are many diseases where the condition can be tuned by modifying the behavior of a particular ion channel. And so even if the ion channel isn’t the primary culprit, by being able to pharmacologically manipulate a particular ion channel, we can provide therapy and treatment for many conditions that afflict us. So I think it is a very good and certainly an important question, and I think that it has a good answer. I think they are unquestionably related to health and disease in humans.
Miller: Let me finish with the last question. Where are you going in the future with your research now? What do you want to do in the next let’s say—five years is infinite time for any scientist—so say within the next four to five years.
MacKinnon: You know me very well. You know my cycle. So I would say in the next four to five years, I want to see the selectivity filter at much higher resolution. I want to see its dynamics, and I want to test this specific idea that one ion talks to another through the structure of the selectivity filter. I think it’s a very interesting feedback chemical device, and I want to test that idea. I would love to see the voltage sensor of a voltage dependent ion channel. So we work hard on that and have a long way to go, but I think that’s going to be a marvelous thing that nature made.
Miller: You’re going to go after another class of stuff.
MacKinnon: And in this case potassium channels, and those are, of course as you know well, the potassium channels that will have a very similar pore to the KCSA potassium channel that we solved. But attached to it, then, there will be the device that allows the pore to open and close in response to changes in membrane voltage. And that’s central to electrical signaling in biology, and I really want to see that structure. Those are the very main questions. There is other work we do with PAS domains and on potassium channel beta subunits of voltage dependent potassium channels, and I view those as feed-ins that tune the voltage sensitive gating. So they’re all part of this project to understand how membrane potential controls the opening and closing of the pore and how different mechanisms then feed in on that to tune it. So that’s what I am thinking about now, and in fact, it’s what keeps me excited when I get up in the morning. And I would say that I would love to be able to do this in five years. I don’t know, I have probably just jinxed myself completely, (laughter) but I would love to be able to do it because I know that I do get itchy. And I also feel like if I don’t really change things in a big way, then I’ll get stuck in my ways, so I probably would then want to do something real different.
Miller: Well, you have a history of doing things different.
MacKinnon: Something that will make me feel completely incompetent and on the edge. That’s what I’ll want to do.
Miller: Some people drive racecars for that feeling.
MacKinnon: Yes. (Laughs.)
Miller: Actually, I have one more question, and maybe it’s a question that won’t work for you because you’re too old now to be an enfant terrible, and you’re too young to be an eminence grise, but could you …
MacKinnon: (Laughing). You’ll have to translate those for me.
Miller: You were one, and you’ll be the other some day. But do you have any kind of advice you would like to offer to scientists emerging in this field, in the field of biomedical research, emerging from their postdocs and facing, what is, I think, to every newly hatched assistant professor in an independent lab a frightening prospect of making contributions and surviving. Not even surviving, but being satisfied, intellectually satisfied, and feeling productive in the research world that we all face now.
MacKinnon: Well, I think we shouldn’t be just shooting to survive. We should be shooting to try to make a difference. But then again I don’t really say that as advice because I think the people who do that, think that way, and the people who don’t, don’t. So you know, just take a risk. Go for it. I think if you crash and burn trying, it’s still going to be better than if you never tried at all.
Key Publications by Roderick MacKinnon
MacKinnon, R. (1991) Determination of the subunit stoichiometry of a voltage-dependent potassium channel. Nature 350: 232–235.
Heginbotham, L., Abramson, T., MacKinnon, R. (1992) A functional connection between the pores of distantly related ion channels as revealed by mutant K+ channels. Science 258: 1152–1155.
Root, M.J. and MacKinnon, R. (1994) Two identical noninteracting sites in an ion channel revealed by proton transfer. Science 265: 1852–1856.
Hidalgo, P. and MacKinnon, R. (1995) Revealing the architecture of a K+ channel pore through mutant cycles with a peptide inhibitor. Science 268: 307–310.
Doyle, D.A., Cabral, J.M., Pfuetzner, R.A., Kuo, A., Gulbis, J.M., Cohen, S.L., Chait, B.T., MacKinnon, R. (1998) The structure of the potassium channel: molecular basis of K+ conduction and selectivity. Science 280: 69–77.
MacKinnon, R., Cohen, S.L., Kuo, A., Lee, A., Chait, B.T. (1998) Structural conservation in prokaryotic and eukaryotic potassium channels. Science 280: 106–109.